
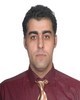
- Open Access
- Authors : B. Boroomandisorkhabi , R. Rahimi
- Paper ID : IJERTV9IS070205
- Volume & Issue : Volume 09, Issue 07 (July 2020)
- Published (First Online): 14-07-2020
- ISSN (Online) : 2278-0181
- Publisher Name : IJERT
- License:
This work is licensed under a Creative Commons Attribution 4.0 International License
Wide Band E-Shaped Nano-Antenna with Asymmetric Hybrid Plasmonic Waveguide
B. Boroomandisorkhabi, R. Rahimi
Department of Electrical and Computer Engineering Missouri University of Science and Technology Rolla, United States of America
AbstractIn this paper, a novel design of end-fire modified wide band E-shaped nano-antenna is introduced with higher gain compare with conventional design at frequency range from 190 THz to 200 THz. An asymmetric hybrid plasmonic waveguide is proposed to feed the designed nano-antenna with less radiation leakage from the waveguide part and high electric field confinement. Overall, far-field radiation of the proposed antenna specifically at higher frequencies is more directive than conventional design.
KeywordsNano-antenna; asymmetrics hybrid plasmonic waveguide
-
INTRODUCTION
Plasmonics show tremendous capability to miniaturize nano-devices specifically nano-antennas and support localized surface plasmon resonance [1]. Plasmonic nano-antennas transfer energy from guided wave modes of an optical waveguide to optical free-space modes and vice versa with high efficiency [2]. However, the bottleneck of plasmonic nano-antennas are their high propagation loss due to their metallic nature. Moreover, in some applications such as optical wireless communications, this kind of antenna should receive optical waves from an Integrated Photonic Circuit (IPC) trough waveguide and radiate it to free space, and vice versa receive the light from free space and couple it to an IPC [3]. Hence, beside high propagation loss, compatibility of plasmonic nano- antennas with fed integrated waveguide is the crucial concern. In purpose of reducing propagation loss and compatibility with IPCs, plasmonic nano-antennas are designed to compatible with dielectric waveguides as feed lines [4].
Hybrid plasmonic patch nano-antennas are known as high efficient nano-antennas, which have the most compatibility with dielectric waveguides compare with other plasmonic designs [5-7]. These types of antenna are inspired by multilayer hybrid plasmonic waveguide, which have moderate trade off between electric field confinement and propagation losses [8- 9]. Simple patch hybrid plasmonic nano-antennas are bidirectional [5] and as a result they have limited applications due to their unwanted radiation. Instead of utilizing simple rectangular patch in palsmonic nano-antennas, E-shaped patch can be used to reach directive radiation pattern [10-11]. In [6], a design of end-fire E-shaped hybrid plasmonic nano-antennas was presented with 8.5 dB gain at 193.5 THz standard optical frequency. However, the gain of the proposed antenna in [6], drops to 5.5 dB at higher frequencies (200 THz). Moreover, in the fabrication process of the antenna, V-shaped silver nano- particles need to be deposed inside slot Silica layer, which imposes extra time and cost consuming etching process. To this end, a new end-fire E-shaped hybrid plasmonic nano-antenna is
proposed in this work to have above 9 dB from 190 THz to 200 THz. Respect to modifications that applied in E-shaped patch in the proposed antenna, far-field radiation pattern of the antenna has less unwanted radiation from sides and back of the antenna compare with conventional design discussed in [6]. Furthermore, the structure of designed antenna is modified to omit etching V-shaped silver nano-particles inside the slab layer.
-
ANTENNA DESIGN
In this work, similar to [6], silicon waveguide with tapered coupler is used to feed E-shaped hybrid plasmonic nano- antenna which is integrated with asymmetric hybrid plasmonic waveguide depicted in Fig. 1. In the proposed design, unlike [6], a single V-shaped silver is placed on top of the silicon nitride (Si3N4) mask layer in the asymmetric hybrid plasmonic waveguide section. The aim of such asymmetric design is confining waves inside the slot layer (10 nm silica layer) and the mask layer of the waveguide and directing majority of waves to the open-ended E-shaped nano-antenna (Theta = 0° chosen as the end-fire). Also, compare to design in [6], field perturbation in the slot layer decreases dramatically because of removing metallic V-shaped particles deposed inside the slot layer. Hence, it is expected unwanted radiation from the waveguide section decreases and as a result gain of the ultimate designed antenna increases specifically at higher frequencies. This claim will be supported by simulation results expressed in Section III.
Moreover, in this work, the conventional design of the E- shaped patch is modified to increase matching between the characteristic impedances of E-shaped patch side wings and free-space impedance (120 ). As discussed in [12-13], the characteristic impedance of side wings is expressed as:
Z0 = 120 / (w/h + 1.393 + 0.667 ln (w/h + 1.444))
Where w is the width of wings and h is the substrate thickness, which is 10 nm for both mask and slot layers. Respect to equation (1), for smaller wings width, the characteristic impedance increases and extreme increase of Z is obtained for w/h < 1.5. Hence, by narrowing sides wings in the proposed nano-antenna, the characteristic impedance increases and become closer to the free space. Accordingly, radiation from wings edge increase and as a result, it is expected antennas gain increases. However, it is worth mentioning, respect to limitation in nano-fabrication, the wings width should not be less than 10 nm for accommodating etching process with conventional wet etching similar to [2].
By deriving optimum wings width respect to the fabrication limits, layers thickness can be determined. To control over layers thickness precisely, among different growth techniques, metal organic chemical vapor deposition (MOCVD) is known as the best choice to control layers thickness in nanometer range [14].
w = 60 nm, antennas gain reduces smoothly from 8.8 dB to
7.9 dB when frequency increases from 190 THz to 200 THz. However, for w = 15 nm, antennas gain reduces only 0.5 dB in 10 THz bandwidth and reach from 9 dB at 190 THz to 8.5 dB at 200 THz. Hence, in the proposed design, w is chosen 15 nm in purpose of reaching high gain antenna with less variation compare with conventional design.
9
8.5
8
Gain(dB)
Gain(dB)
7.5
7
6.5
6
Conventional Design (Ref [6]) Proposed Design
Fig. 1. Designed modified E-shaped nano-antenna with asymmetric hybrid plasmonic waveguide.
-
SIMULATION RESULTS
In this Section, simulations are prepared by employing CST Microwave Studio® to study matching and radiation characteristic of proposed E-shaped nano-antenna with asymmetric hybrid plasmonic waveguide. As discussed in Section II, modifying asymmetric hybrid plasmonic waveguide could improve radiation efficiency by reduction of unwanted radiation from the waveguide. To this end, instead of locating three V-shaped silver nano-particles inside the silica slab layer, a single V-shaped silver particle is deposed on top of the slot and mask layers to suppress unwanted radiation from top of the waveguide and direct waves toward open-ended of the antenna (Theta = 0°). Hence, the gain of the antenna increases compares to conventional design in [6] at frequencies higher than 192 THz. In Fig. 2, simulated gain of the E-shaped antenna is shown when proposed hybrid plasmonic waveguide and conventional plasmonic waveguide discussed in [6] are employed. For accurate comparison, the shape and dimension of E-shaped patch nano-antenna for both simulations keep fixed and equal to conventional design. As seen, the gain of the antennain conventional design drops sharply from 8.8 dB at 190 THz to 5.5 dB at 200 THz. On The other hand, at this frequency range, by employing the proposed hybrid plasmonic waveguide, antennas gain varied between 8.3 dB to 7.5 dB.
To increase antennas gain, the antennas impedance need to be adjusted to be more close to free-space impedance (377 ohm). As discussed in Section II, by reducing the width of side wings, the characteristic impedance increases and closer to 377 ohms. To this end, for 3 different wings width, antennas gain is calculated numerically by employing CST software. In Fig.- 3, antennas gain versus frequency s shown as a function of wing width (w). The initial wing width is chosen 128 nm same as the conventional antennas wings width in [6]. Also, for smaller with 60 nm and 15 nm, antennas gain is calculated an as shown for smaller width, gain of the antenna increases. For
5.5
190 192 194 196 198 200
Frequency (THz)
Fig. 2. Simulated gain of the E-shaped antenna when proposed hybrid plasmonic waveguide and conventional plasmonic waveguide discussed in [6] are employed.
9.5
9
8.5
Gain(dB)
Gain(dB)
8
7.5
7
Conventional Design (Ref [6]) Proposed Design, w = 128 nm Proposed Design, w = 60 nm Proposed Design, w = 15 nm
Conventional Design (Ref [6]) Proposed Design, w = 128 nm Proposed Design, w = 60 nm Proposed Design, w = 15 nm
6.5
6
5.5
190 192 194 196 198 200
Frequency (THz)
Fig. 3. Conventional and proposed antennas gain versus frequency as a function of wing width (w).
Next, effect of metallic V-shaped particles thickness on antennas radiation pattern and matching is studied. In Fig. 4, simulated reflection coefficient of the proposed antenna in terms of frequency is depicted for 3 different metallic layer thickness. For simulated thickness equals 10 nm and 20 nm, reflection coefficient is less than -10 dB (acceptable matching for antennas in passband). However, for thicker metallic layer,
reflection coefficient decreases more and consequently impedance matching of the antenna increases. On the other hand, directivity at the front end of the antenna reduces by deviating main lobe the far-field radiation pattern of the antenna from Theta = 0. To show destructive effect of thicker metallic layer on antennas directivity, far-field radiation pattern of the antenna at H-plane (Phi = 90°) is depicted in Fig. 5 for metallic layer witp0 nm, 20 nm and 50 nm thickness. As seen, by increasing metallic layers thickness from 10 nm to 50 nm, main lobe direction deviates from Theta = 0 to Theta = 330. Hence, the optimum metallic layers thickness is 10 nm to have end fire antenna.
Hm = 10 nm Hm = 20 nm
Hm = 50 nm
Hm = 10 nm Hm = 20 nm
Hm = 50 nm
-8.5
-9
Reflection Coefficient(dB)
Reflection Coefficient(dB)
-9.5
-10
-10.5
-11
-11.5
-12
190 192 194 196 198 200
Frequency (THz)
Fig. 4. Reflection coefficient of the proposed antenna in terms of frequency for 3 different metallic layer thickness.
Fig. 5. Far-field radiation pattern of the antenna at H-plane (Phi = 90°) for metallic layer with 10 nm, 20 nm and 50 nm thickness.
Finally, far-field radiation pattern of the proposed antenna with optimum dimensions (shown in Fig. 1) at E-plane (Phi = 0°) is depicted in Fig. 6 and compared with far-field radiation
pattern of the conventional design [6] at 193.5 THz (Fig. 6 (a)) and 200 THz (Fig. 6 (b)). As seen in Fig. 6 (a), the directivity of the proposed antenna and conventional design, is approximately same but the proposed antenna has less back lobe compare with conventional design. At 200 THz, antennas directivity for the proposed design is higher than conventional design. Moreover, back lobe level is around 6 dB less in the proposed design compare with conventional one.
Fig. 6. (a) far-field radiation pattern of the conventional design at 193.5 THz.
(b) far-field radiation pattern of the conventional design at 200 THz.
-
CONCLUSION
In this work, modified E-shaped nano-antenna is proposed, which is fed by novel asymmetric hybrid plasmonic waveguide. Unlike conventional design discussed in [6], the new designed has less than 1 dB gain variation from the range between 190 THz and 200 THz. Moreover, back lobe and side lobe level of the proposed antenna with integrated waveguide can reach to 5 dB less than conventional one. Finally, due to modification applied to the waveguide, etching process for
shaping nanoparticles is removed from the fabrication process, which cause less fabrications complexity
REFERENCES
-
N. Large, M. Abb, J. Aizpurua, and Otto L. Muskens, Photoconductively Loaded Plasmonic Nanoantenna as Building Block for Ultracompact Optical Switches, Nano letters, Vol. 10, No. 5, 1741- 1746, 2010.
-
Z. Manzoor, M. A. Panahi, and A. Pak, Multicomponent-multilayer hybrid plasmonic leaky-wave optical antenna with improved directivity and matching, Terahertz, RF, Millimeter, and Submillimeter-Wave Technology and Applications XI. Vol. 10531, International Society for Optics and Photonics, 2018.
-
L. Yousefi, Highly directive hybrid plasmonic leaky wave optical nano- antenna, Progress In Electromagnetics Research Letters, Vol. 50, 85- 90, 2014.
-
Z. Manzoor, M. A. Panahi, and A. Pak, Hybrid Wedge-integrated Plasmonic-photonic Waveguide, 2019 United States National Committee of URSI National Radio Science Meeting (USNC-URSI NRSM). IEEE, 2019.
-
L. Yousefi, A. C. Foster, Waveguide-fed optical hybrid plasmonic patch nano antenna, Opt. Exp., vol. 20, No. 16, 18326-18335, 2012.
-
Z. Manzoor, M. A. Panahi, and A. Pak, E-shaped Nano-antenna with Asymmetric Integrated Dielectric-plasmonic Waveguide, 2019 IEEE International Symposium on Antennas and Propagation and USNC- URSI Radio Science Meeting. IEEE, 2019.
-
P. Sharma, and V. Dinesh Kumar, Multilayer Hybrid Plasmonic Nano Patch Antenna, Plasmonics, Vol. 14, No. 2, 435-440, 2019.
-
Z. Manzoor, A. Mirala, A. Pak, and M. A. Panahi, Low loss semiMIM hybrid plasmonic waveguide with high electric field confinement, Microwave and Optical Technology Letters, Vol. 61, No. 11, 2557- 2564, 2019.
-
R. F. Oulton, V. J. Sorger, D. A. Genov, D. F. P. Pile, A hybrid plasmonic waveguide for subwavelength confinement and long-range propagation, nature photonics, Vol. 2, No. 8, 496-500, 2008.
-
M. H. El Sherif, M. H. Bakr, and E. A. Soliman, E-shaped wideband plasmonic nantennas with linear and dual-linear polarizations, Photonics Research, Vol. 3, No. 4, 140-145, 2015.
-
B. Han, X. Li, C. Sui, J. Diao, X. Jing, and Z. Hong, Analog of electromagnetically induced transparency in an E-shaped all-dielectric metasurface based on toroidal dipolar response, Optical Materials Express, Vol. 8, No. 8, 2197-2207, 2018.
-
Z. Manzoor, and G. Moradi, Optimization of Impedance Bandwidth of a Stacked Microstrip Patch Antenna with the Shape of Parasitic Patch's Slots, Applied Computational Electromagnetics Society Journal, Vol. 30, No. 9, 1014-1018, 2015.
-
V. K. Pandey, and B. R. Vishvakarma, Analysis of an Eshaped patch antenna, Microwave and Optical Technology Letters, Vol. 49, No. 1, 4- 7, 2007.
-
V. Saravade, Z. Manzoor, A. Corda, C. Zhou, and N. Lu, Nickel doping in zinc oxide by MOCVD: structural and optical properties, Quantum Sensing and Nano Electronics and Photonics XVII., Vol. 11288, International Society for Optics and Photonics, 2020.