
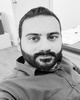
- Open Access
- Authors : Hassan I. Alhammad , Ibrahim O. Habiballah
- Paper ID : IJERTV10IS120012
- Volume & Issue : Volume 10, Issue 12 (December 2021)
- Published (First Online): 22-12-2021
- ISSN (Online) : 2278-0181
- Publisher Name : IJERT
- License:
This work is licensed under a Creative Commons Attribution 4.0 International License
Fault Ride Through for Solar Photovoltaic and Wind-Turbine Integrated Distribution Network: A Systematic Review
Hassan I. Alhammad1,* Ibrahim O. Habiballap
Department of Electrical Engineering , King Fahd University of Petroleum and Minerals1 Dhahran, Saudi Arabia 31261
Abstract: The purpose of this paper is to provide a systemic review on fault ride through (FRT) capabilities. As there are multiple types of power system faults, where each type has different effect on the power quality, faults types along with their effects on the power quality are firstly highlighted for better understanding of the FRT capabilities. The FRT techniques along with its types are then introduced. Furthermore, the most common renewable energy sources where FRT techniques are implemented are discussed. Recent Improvement methods for FRT are highlighted and summarized. The protection devices used to detect and isolate faults are lastly introduced, where the paper is then concluded.
Index Terms: Power System Faults, fault ride through, fault detection, solar photovoltaic systems, wind turbines
-
INTRODUCTION
An electric power system is a multifaceted power network referred to as a grid and consists of generation plants, transmission lines, and distribution networks that are spread over a large geographical area. The design configuration and the operation characteristics of an electric power system is what determine the complexity of the system. A fault in such a complex power system is a disturbance of the normal power flow from the source to the load. Faults mostly occur within the transmission lines causing an insulation failure of the electrical components due to the damage caused by mechanical issues or environmental reasons such as lightning or a fall of a tree on the transmission line [1], [2]. The insulation damage exposes generators, transformers, transmission lines, cables, etc. to extreme heating, which can eventually lead to fire or explosion. The power system; as a result, can completely shut down due to the disruptive effect of power system stability.
The increasing demand for energy in the modern-day world, in presence of restricted use of conventional energy, shifted us towards renewable energy (REN). As a result, the integration of REN sources has significantly grown which led to the facilitation of different operational aspects such as protection, stability, reliability and power quality [3]. The 2030 REN target for the selected countries is given in Table 1.1.
Country
Target Percentage of REN Generation
Target year
New Zealand
90% of energy production is from REN
2025
Saudi Arabia
80% of energy production is from REN
2030
France
32% of energy production is from REN
2030
Turkey
30% of energy production is from REN
2030
Japan
22%24% of energy production is from REN
2030
South Korea
11% of energy production is from REN
2030
South Africa
10% of energy production is from REN
2030
Table 1.1 2030 renewable energy target for selected countries
Based on the connection complexity of power generating units (such as solar photovoltaic (PV) systems, wind turbines (WTs), diesel generators, etc.), the transmission system operators (TSOs) establish a set of operative regulations called the grid code in order to coordinate the integration of these generation units to the grid. Grid codes are developed based on international standards such as the American National Standards Institute (ANSI) and the International Electrotechnical Commission (IEC). However, grid codes may be modified by TSOs based on the local network requirements. The aim of the grid code is to maintain the stability of the grid by regulating the voltage and frequency. As a result, the grid model during design stage may be subject to changes due to grid codes requirements [4].
Fault ride through (FRT) is a key component of the grid code, and its properties have an impact on the performance and rating of power system equipment. FRT specifies the capabilities required for a generation unit to ride through a voltage or frequency disturbance in order to prevent the loss of that generation unit during and in post-fault conditions. In order to set the FRTs requirements, the fault type shall be determined as each type of fault has a different effect on the voltage and frequency of the system [5].
In [6], faults in a three-phase system are introduced where a unified fault matrix method is used for calculating open and short circuit faults which shows a complete duality between open and short circuit faults. [7][9] give a comprehensive analysis about the FRT techniques, including LVRT, ZVRT and HVRT, and controllers which already have been implemented in the grid code of 38 countries for renewable energy sources. [10] discusses current injection methods related to FRT techniques for GCPV systems, while [11] reviewed the state-of-the-art FRT enhancement methods for GCPV systems.
Hitherto, many methods are invented to detect faults within different parts of the power system. In [12], [13] a survey on various practices for fault classification in transmission lines is provided. The survey introduces old as well as newly developed approaches in fault classification. [14] proposes another technique to detect faults during power swing. However, it comprises the signals of both voltages and currents as one quantity. Since the proposed fault detector works with fixed thresholds, it is claimed to be setting-free, meaning revising from one system to another system is not needed. [15] proposes an intelligent protection scheme, which eliminates the need to install costly limiters of current fault. This protection scheme utilizes communication capabilities specified in IEC 61850 to design intelligent cascading switching actions to isolate the defective part of the system. [16], [17] proposes intelligent artificial methods (include fuzzy logic, artificial neural network (ANN), genetic algorithm (GA), support vector machine (SVM) and matching approach) that are based on wavelet theory to detect faults within a distribution network. This method is intended to provide a mean of islanding detection, which refers to the situation in which a main power source forms microgrids if it is disconnected without stopping the distributed renewable sources.
The structure of this paper is as follows. In section II, types of power system faults and their effects on power quality are discussed. In section III, FRT capabilities along with their types are introduced. Section IV represents the most common REN systems that FRT techniques are used with while section V highlights improvement approaches for FRT. Section VI represents the protection devices used to detect and isolate faults. Section VII concludes this paper.
-
TYPES OF FAULTS
Faults in power systems are divided into open circuit (also called series) faults and short circuit (also called shunt) faults. Faults shall stimulate the switching of the protection devices. Therefore, faults occur at the terminals of either active or passive electrical equipment. Active electrical equipments include synchronous and asynchronous machines or grid equvalents. Passive electrical equipment include transformers, transmission lines, or loads
-
Open Circuit Faults:
Open circuit faults are characterized by an increase in voltage and frequency and a decrease in current in the faulted phase. Therefore, they can be detected by monitoring the voltage of each phase. If the voltage value rises, it means that an open- circuit fault happened. Open circuit faults cause cessation of current flow and they rarely occurs.
-
Short Circuit Faults:
Whenever there is a short circuit fault, the voltage and frequency will decrease while the current will increase in the faulted phase. Accordingly, they can be detected by the observation of the current of each phase. If there is an increase in the current value, it highlights the initialization of a short circuit fault.
Short circuit faults are the most hazardous as the current is increased to a lot more (e.g. 10 times more) than the nominal current of the instrumentation. Short circuit fault may take an arc form on overhead transmission lines causing the conductor to burn and break if not timely cleared. Short circuit faults may also turn the faulted phase into loads in interconnected systems and that is because of the large decrease in voltage and frequency in those faulted phases. Accordingly, induction and synchronous motors may feed the fault during a short circuit fault which worsens the situation even more. Shorts are commonly occurring and there are two main types of short circuit faults, namely: symmetrical faults and unsymmetrical faults.
-
Symmetrical Faults:
A symmetrical fault is balanced and it affects all phases equally as shown in Figure 2.1, meaning the voltage drops of all the three-phase is equal and has the same magnitude, which is proportional to the severity of the faults. A symmetrical fault is the most severe because it involves all three phases and roughly 5 % of faults involved in a three-phase system are symmetrical.
Figure 2.1 Scheme of symmetrical fault
-
Asymmetrical Faults:
An asymmetrical fault is unbalanced and it affects each phase separately, meaning the voltage drop of each phase is uneven and has different magnitude depending on the nature of the fault. An asymmetrical fault can occur as a line-to-ground (L-G) fault, line-to-line (L-L) fault, or double-line-to-ground (L-L-G) fault. Asymmetrical faults are difficult to analyze and
almost 95% of faults involved in a three-phase system are asymmetrical. The subsections below will provide additional details about the types of asymmetrical faults:
-
Line-to-ground Fault:
A line-to-ground (L-G) fault is caused by a conductor making contact with a grounded structure and it is the most common. 70% of the entire faults are found to be L-G faults. For an L-G faults to occur as shown in Figure 2.2, the condition is: I = 0, I = 0 and V = 0
Figure 2.2 Scheme of L-G fault
-
Line-to-line Fault:
If one phase touches another phase, it causes a line-to-line (L-L) fault. About 15% of all faults are found in L-L faults.
For the L-L fault to occur as shown in Figure 2.3, the condition is: I = 0, I = 0, V = 0 and V = 0
Figure 2.3 Scheme of L-L fault
-
Double-line-to-Ground Fault:
When two phases come in contact with a ground, it leads to a double-line-to-ground (L-L-G) fault. 10% of all faults fall under this type. For the L-L-G fault, shown in Figure 2.4, to occur, the boundary condition is: I = 0, V = 0 and V = 0
Figure 2.4 Scheme of L-L-G fault
-
-
-
-
Fault Ride Through
Continuity of electric power supply is essential; therefore, issues that might cause a loss of a generation unit are unsolicited. The purpose of preventing a generation unit loss using FRT techniques is as much as economically important as it is technically. A voltage-time or frequency-time diagram called the FRT diagram is used to demonstrate the essential requirements a generation unit shall meet to ride through a voltage or frequency disturbance. The FRT diagram may be different among countries as the grid codes differ.
During a fault, the grid code require the generation unit to stay connected to the grid within a certain range of voltage and frequency. Accordingly, the setting of the protection devices strongly relies on FRT requirements, which may require the generation units to generate maximum reactive power without violating the transient rating limits. The parameters involved in defining the FRT requirements include the follow:
-
network design
-
generator type
-
fault type and amplitude (commonly short circuit faults)
-
fault occurrence impedance
-
fault clearance period
-
power control procedure
The effects of the symmetrical and asymmetrical short circuit faults on the threephase voltage magnitudes vary. Consequently, they affect requirement of the FRT differently. Furthermore, the location of the generation units and other components is crucial in the application of the FRT.
-
Voltage Ride Through
During a fault, changes will occur to the systems voltage, current, and frequency which can lead to the disconnection of some generation units from the grid. This reduces the reliability of the power system. Therefore, TSOs, in presence of high integration of REN sources, require generation units to have LVRT capabilities or even ZVRT capabilities. In some cases, TSOs will require HVRT capability as well.
A LVRT capability curve is displayed in Figure 3.1. The voltage of a generation unit operating in normal condition (Vn) is as demonstrated in area A. During a symmetrical fault or an asymmetrical L-L or L-L-G fault, which occurs at the time interval (t0), the generating unit experiences a voltage sag (Vl). The LVRT allows the generating unit to remain connected to the grid by withstanding the voltage drop for a certain time (represented in area B). When the voltage level reaches at area C, the generating unit should disconnect from the power system. The voltage is regulated back to Vr when the fault is cleared after t2. This LVRT capability ensures fast post-fault recovery and stability for the system.
Figure 3.1 Lowvoltage ride through (LVRT) curve
Furthermore, the occurrence of asymmetrical L-G fault causes a voltage swell. When a voltage swell occurs, the generating withstands this condition through a HVRT capability. A HVRT capability curve is displayed in Figure 3.2.
Figure 3.2 Highvoltage ride through (HVRT) curve
-
Frequency Ride Through
Frequency ride through (FrRT) capability is another type of FRT. This type ensures that the operation of generation plants are governed by strict guidelines. Hence, the generation operations are not disturbed by sudden frequencychanging conditions.
The instantaneous balance of load and generation determines the grid interconnection frequency. Therefore, a loss of a transmission line, or a generation unit or load disturbs the frequency. Such a loss can unbalance between the load and generation causing under-frequency or over-frequency conditions.
A loss of generation causes an under-frequency condition while a loss of load cases a high-frequency condition. TSOs; therefore, require utilities to apply load-shedding policies that automatically respond to under-frequency events. On the other hand, TSOs also require that bulk generators to have under-frequency ride-through (UFrRT) capabilities that extends beyond the
lowest frequency of these load-shedding policies as well as high-frequency ride-through (HFrRT) capabilities that extends beyond the highest frequency of these load-shedding policies.
-
-
FRT FOR RENEWABLE SOURCES
Increased integration of REN sources makes TSOs more conscious towards them during faults as they have significant effects on the stability of te power system [18][20]. Because of the large integration of REN sources; for example, FRT- related grid codes in the United Kingdom, Japan, Germany, and most recently in the unified European Network of Transmission System Operators for Electricity (ENTSOE) network contain multistage and complex rules with many specifications. Consequently, the FRT technical requirements in the grid codes should consider the integration percentage of REN sources.
Solar PV systems and WTs are the most REN sources exposed to grid faults as they are the most common integrated into a conventional grid; hence, they play a big role in developing the grid codes. One of the major challenges in maintaining solar PVs and WTs connected to the grid during faults is the lack of necessary requirements such as the automated voltage regulator (AVR).
-
FRT for Solar Photovoltaic (PV)
GCPV systems typically operate in balanced conditions, with ideal load voltages and currents that are within the inverter's operational limits. The occurrence of a grid fault interrupts the current flow of the inverter for a few milliseconds, resulting in low impedance and short circuit faults (categorized by current increase, and voltage and frequency decrease in the faulted phases). This makes the GCPV systems in an unbalanced condition. The FRT enables the PV system to remain connected to the grid when the voltage reduces through LVRT capabilities. Even if the voltage is reduced to zero, the PV system should remain connected to the grid through ZVRT capabilities. During a fault condition, the PV system generates reactive power (analogous to the excitation increase in conventional generators) in order to increase the grids voltage. When a disturbance is cleared, PV systems must promptly generate pre-fault active power. A common configuration of a GCPV system that has a FRT capability is shown in Figure 4.1.
Figure 4.1 Configuration of grid-connected photovoltaic (GCPV) system
Every GCPV system is composed of three primary components that are: PV array, DC-to-DC converter, and DC-to- AC inverter. The inverter is critical to GCPVs since it controls the voltage as well as the active and reactive powers in accordance with grid requirements. The proposed control scheme forces the inverter to remain connected to the grid during 50% and 100% voltage sag by separating the grid voltages sequences. The positive sequence is fed to a phase-locked loop (PLL) where a feed-forward loop (FFL) and current limiters are augmented in order to maintain stability [21]. The control scheme accordingly supports the inverter to ride through the fault; however, the swinging of the reactive and reactive powers is increased as they are dependent on the direct-axis (d-axis) and quadrature-axis (q-axis) algorithm. Therefore, the current and voltage are initially measured and used to calculate the d-axis and q-axis voltages (Vd and Vq) and currents (Id and Iq) from
which the reference d-axis and q-axis currents (I* and I*) and voltages (V* and V*) are determined in order to satisfy the LVRT
d q d q
requirements as shown in Equation 1 and 2.
V* (t) = (K + Ki ) [ I (t)-I*(t)] (1)
d p S d d
V* (t)= (K + Ki ) [I*(t)- I (t)] (2)
q p S q q
Where (K + Ki ) is the application of a PI transfer function on the error signal e and K K
are PI controller
p S p i
parameters. The reference voltages in Equation 1 and 2 then fed to the inverters control gate circuit to operate the LVFRT or ZVRT capabilities. Similar approach is followed for the negative, positive and the zero sequence.
-
FRT for Wind Turbines
WTs operate in balanced conditions, similar to PV systems, with ideal load voltages and currents that are within the inverter's operational limits. When a fault occurs, the WTs are required to remain connected to the grid through LVTR capabilities. The LVRT capabilities for WTs are established based on the topology and control strategy of the WTs circuit. The LVRT capabilities for WTs normally reduce reactive drawl and even generate reactive power during a faulty condition. When the fault is cleared, the WTs promptly produce active power when possible. A common configuration of a WT that has a FRT capability is shown in Figure 4.2.
Figure 4.2 Configuration of wind turbine (WT)
The objective of the inverter is to balance the 3-phase power that is provided by the machine-side rectifier by shaping the output current. Failing to achieve this objective will cause the power to be unbalanced; thus, resulting in variation of the DC- link voltage. The current and voltage are then used to calculate the d-axis and q-axis voltages (Vd and Vq) and currents
(Id and Iq) from which the reference d-axis and q-axis currents (I* and I*) and voltages (V* and V*) are determined in order to
d q d q
satisfy the LVRT requirements as shown in Equation 3 and 4.
V (t) = V (t) + (K + Ki ) [I (t) I (t)] + L (K + Ki ) [I (t) I (t)] (3)
d d p S d d c p S d d
V (t) = V (t) + (K + Ki ) [I (t) I (t)] L (K + Ki ) [I (t) I (t)] (4)
q q p S q q c p1 S q q
Where (K + Ki ) is the application of a PI transfer function on the error signal e and K K
are PI controller
p S p i
parameters. The reference voltages in Equation 3 and 4 then fed to the inverters control gate circuit to operate the LVFRT or ZVRT capabilities. Similar approach is followed for the negative, positive and the zero sequence.
-
-
FRT IMPROVEMENT METHODS
Many methods have been proposed to improve the FRT. These methods can be classified as passive or active methods as highlighted Table 5.1. The implementation of passive methods depends on using auxiliary devices while active methods do not. All of these methods have been theoretically validated, and experimental findings show that they perform well.
Approach
Advantages
Passive Methods
Blade Pitch Angle Control
Crowbar Method
Energy Capacitor System
Energy Storage System
Active Methods
Generator Side Converter Control
AC Grid Side Converter Control
-
Allow power reduction
-
Increases energy capture
-
Generator and converter fault protection
-
Active-reactive power regulation
-
Allows grid voltage regulation
-
Costeffective
-
Over-voltage protection
-
Minimum impact on rotor current
-
Improves energy saving
-
Generator and converter fault protection
-
Energy market regulation
-
Generator and converter fault protection
-
Allows grid voltage regulation
Table 5.1 Fault ride through (FRT) improvement approaches
-
-
FAULTS DETECTION
The complexity in the configuration of an electric grid and the urgency of power supply make a fault unwanted even if there are FRT capabilities within the grid. Faults are expected in power systems; however, they have to be timely detected and clear. Transmission lines are the first line to ensure the integrity of the grid; thus, TSOs must always ensure the networks reliability and stability. When a fault occurs, the affected branch of the power network should be automatically isolated. For this purpose, many protection devices are used such as fuses, circuit breakers, relays, and instrument transformers to isolate faults. The function of these protection devices is as follows:
-
Fuse: it is an electrical safety device that provides overcurrent protection. It is essentially composed f a metal wire or strip that melts as excessive current flows through it; thereby it interrupts the current flow
-
Circuit breaker: it is an electrical safety switch that is automatically operated to protect against overload or short circuit damage. It detects a faulty condition and interrupts the current flow by isolating the faulted branch in an electrical circuit
-
Relay: it is an electrical safety switch that commands a circuit breaker to trip when it senses a fault, in order to isolate faulted branches of the electrical circuit
-
Instrument transformer: it is an electrical measuring device (mainly coupled with a relay) that protects a power network substation against faults at high-level applications (e.g. high voltage or high current application). If a fault occurs, it transforms the voltage or current level down and isolates it
If protection devices timely and reliably operate, the detection of any deterioration in the system status will be easily identified. It will; accordingly, play a conclusive role to protect the operation of the grid. Otherwise, system crashes will be accelerated, hence, large-scale and long-time power blackout will occur. One of many serious events triggered by a gird fault is the large-scale blackout of the America-Canada power network that occurred on August 14, 2003. It was a result of an overload fault, which caused the removal of four connection lines between the cities of Cleveland and Akron in Ohio.
-
-
CONCLUSION
A fault is a disturbance that affects the normal flow of current to the load. In this paper a systematic review of FRT capabilities is provided discussing FTR types and the most common REN system that FRT techniques are used with. For a better understanding of the FRT capabilities, types of power system faults along with their effects on power quality are introduced and discussed. The paper is finally concluded by introducing the protection devices used to detect faults.
Integration of REN sources into the grid to replace conventional generators has dramatically increased; as a result, the total inertia of the power system is reduced. This integration of REN sources makes the stability of the power system during faults a critical concern. In this regard, the FRT technical requirements will gain more attention among researchers in universities and industries
ACKNOWLEDGEMENT
The author would like to acknowledge the resourcing support facilitated from the Deanship of Research (DSR) at King Fahd University of Petroleum and Minerals.
REFERENCES
-
H. Zhang, W. Xiang, W. Lin, and J. Wen, Grid Forming Converters in Renewable Energy Sources Dominated Power Grid: Control Strategy , Stability , Application , and Challenges, vol. 9, no. 6, pp. 12391256, 2021, doi: 10.35833/MPCE.2021.000257.
-
J. He et al., International Journal of Electrical Power and Energy Systems The improved fault location method based on natural frequency in MMC- HVDC grid by combining FFT and MUSIC algorithms , no. November, 2021, doi: 10.1016/j.ijepes.2021.107816.
-
H. I. Alhammad, K. A. Khan, O. F. Konash, and M. Khalid, Deployment of Battery Energy Storage System in a Renewable Integrated Distribution Network Based on Long-Term Load Expansion, 2021 31st Australasian Universities Power Engineering Conference (AUPEC), 2021, pp. 1-6, doi: 10.1109/AUPEC52110.2021.9597750.
-
L. Meegahapola, M. Datta, I. Nutkani, and J. Conroy, Role of fault ride-through strategies for power grids with 100% power electronic-interfaced distributed renewable energy resources, Wiley Interdiscip. Rev. Energy Environ., vol. 7, no. 4, pp. 124, 2018, doi: 10.1002/wene.292.
-
G. Angala Parameswari and H. Habeebullah Sait, A comprehensive review of fault ride-through capability of wind turbines with grid-connected doubly fed induction generator, Int. Trans. Electr. Energy Syst., vol. 30, no. 8, pp. 130, 2020, doi: 10.1002/2050-7038.12395.
-
L. Hofmann, Unified fault matrix method for the calculation of shunt and series faults in power systems with complete duality of shunt and series fault matrices, Electr. Power Syst. Res., vol. 189, no. July, p. 106556, 2020, doi: 10.1016/j.epsr.2020.106556.
-
R. M. Kamel, Three fault ride through controllers for wind systems running in isolated micro-grid and Effects of fault type on their performance: A review and comparative study, Renew. Sustain. Energy Rev., vol. 37, pp. 698714, 2014, doi: 10.1016/j.rser.2014.05.065.
-
M. T. Hagh and T. Khalili, A review of fault ride through of PV and wind renewable energies in grid codes, Int. J. Energy Res., vol. 43, no. 4, pp. 13421356, 2019, doi: 10.1002/er.4247.
-
D. Eltigani and S. Masri, Challenges of integrating renewable energy sources to smart grids: A review, Renew. Sustain. Energy Rev., vol. 52, pp. 770780, 2015, doi: 10.1016/j.rser.2015.07.140.
-
Z. Hassan, A. Amir, J. Selvaraj, and N. A. Rahim, A review on current injection techniques for low-voltage ride-through and grid fault conditions in grid-connected photovoltaic system, Sol. Energy, vol. 207, no. June, pp. 851873, 2020, doi: 10.1016/j.solener.2020.06.085.
-
A. Q. Al-Shetwi, M. Z. Sujod, F. Blaabjerg, and Y. Yang, Fault ride-through control of grid-connected photovoltaic power plants: A review, Sol. Energy, vol. 180, no. January, pp. 340350, 2019, doi: 10.1016/j.solener.2019.01.032.
-
A. Prasad, J. Belwin Edward, and K. Ravi, A review on fault classification methodologies in power transmission systems: PartI, J. Electr. Syst. Inf. Technol., vol. 5, no. 1, pp. 4860, 2018, doi: 10.1016/j.jesit.2017.01.004.
-
A. Prasad, J. Belwin Edward, and K. Ravi, A review on fault classification methodologies in power transmission systems: Part-II, J. Electr. Syst. Inf. Technol., vol. 5, no. 1, pp. 6167, May 2018, doi: 10.1016/J.JESIT.2016.10.003.
-
B. Mahamedi and J. E. Fletcher, Setting-free method for detection of asymmetrical faults during power swings, Electr. Power Syst. Res., vol. 181, no. January, p. 106177, 2020, doi: 10.1016/j.epsr.2019.106177.
-
M. Mahmoudian Esfahani and O. Mohammed, An intelligent protection scheme to deal with extreme fault currents in smart power systems, Int. J. Electr. Power Energy Syst., vol. 115, no. July 2019, p. 105434, 2020, doi: 10.1016/j.ijepes.2019.105434.
-
C. Darab, R. Tarnovan, A. Turcu, and C. Martineac, Artificial Intelligence Techniques for Fault Location and Detection in Distributed Generation Power Systems, Proc. 2019 8th Int. Conf. Mod. Power Syst. MPS 2019, pp. 14, 2019, doi: 10.1109/MPS.2019.8759662.
-
R. Binns, S. Juma Al-Mamari, and M. Salehifar, Instantaneous Faults Detection in Power System, 2018 20th Int. Middle East Power Syst. Conf. MEPCON 2018 – Proc., no. 1, pp. 9096, 2019, doi: 10.1109/MEPCON.2018.8635170.
-
H. I. Alhammad, M. F. Alsada, K. A. Khan, and M. Khalid, A voltage sensitivity framework for optimal allocation of battery energy storage systems, IEEE Green Technol. Conf., vol. 2021-April, pp. 558562, 2021, doi: 10.1109/GreenTech48523.2021.00093.
-
K. A. Khan and M. Khalid, A reactive power compensation strategy in radial distribution network with high PV penetration, 8th Int. Conf. Renew. Energy Res. Appl. ICRERA 2019, pp. 434438, 2019, doi: 10.1109/ICRERA47325.2019.8997110.
-
H. I. Alhammad, K. A. Khan, F. Alismail, and M. Khalid, Capacity Optimization and Optimal Placement of Battery Energy Storage System for Solar PV Integrated Power Network, 2021 IEEE Energy Conversion Congress and Exposition (ECCE), 2021, pp. 847-852, doi: 10.1109/ECCE47101.2021.9595426.
-
C. H. Ng, L. Ran, and J. Bumby, Unbalanced-grid-fault ride-through control for a wind turbine inverter, IEEE Trans. Ind. Appl., vol. 44, no. 3, pp. 845856, 2008, doi: 10.1109/TIA.2008.921429.