
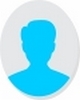
- Open Access
- Authors : Sameh Yehia , Mayadah W. Falah , Badr Abouzeid
- Paper ID : IJERTV11IS030042
- Volume & Issue : Volume 11, Issue 03 (March 2022)
- Published (First Online): 21-03-2022
- ISSN (Online) : 2278-0181
- Publisher Name : IJERT
- License:
This work is licensed under a Creative Commons Attribution 4.0 International License
Flexural Behavior of Lightweight Polymer Concrete Slab Reinforced with Glass Fiber Reinforced Polymer Bars
Sameh Yehia1,Mayadah W. Falap, and, Badr Abouzeid3
1 Associate Professor, Faculty of Engineering, Suez University, Suez, Egypt.
2 Department of Building & Construction Technology Engineering, Al-mustaqbal University College, 51001 Hillah, Babil, Iraq.
3 Assistant Professor, Faculty of Engineering, Suez University, Suez, Egypt.
Abstract:- The flexural behavior of polymer impregnated lightweight concrete reinforced with fiberglass-reinforced polymer (GFRP) bars was examined in this research. The primary distinction between lightweight and regular concrete is the reduced density mass. Using lightweight concrete minimizes the structure's dead load, allowing the structural designer to shrink the size of the column, footing, and other load-bearing elements. Because lightweight concrete is non-structural, polyester is employed in structural engineering to create a new generation of lightweight concrete impregnated with polymer. In addition, to resist flexural and tensile stress, glass fiber reinforced polymer (GFRP) is used in slabs.
Keywords: Recycling, External Strengthening, GFRP, Green Concrete, Lightweight Concrete, Fibrous Concrete.
-
INTRODUCTION:
For many decades, Lightweight concrete is considered non-structural concrete because the aggregate utilized is of a special nature. Compared to conventional weight concrete, which has a density of 2240 to 2400 kg/m3, structural lightweight aggregate concrete (SLWAC) has an in-place unit weight of 1440 to 1840 kg/m3. The cylinder compressive strength should be greater than 17.0 MPa for structural applications. In most cases, the marginally higher cost of the SLWAC is offset by size reduction of structural elements, less reinforcing steel, and reduction in concrete volume, Resulting in lower overall cost [1][7]. Lightweight aggregate plays the primary role in reducing the density of concrete, which helps to reduce the own weight of the slab and reduce the dead load in general on the structure, especially on the building foundations. The use of lightweight porous concrete is a new approach to obtaining small concrete density, but this is at the expense of concrete strength. Polymers are the best solution to overcome the weakness of concrete strengths. Hence, they can combine the concrete components, which helps integrate the molecules to improve concrete behavior [8], [9]. Despite the additional cost of utilizing polymers and their significant weakness in resisting fire and heat, it is considered the best choice for submarine buildings that are exposed to steel rust. To complement the innovative idea in this research, glass fiber reinforced polymer (GFRP) bars have been utilized as the main reinforcement for studied slabs. Utilizing these bars enhances the bond between these bars and the polymer material because these bars are made of the same material, which improves the behavior of the composite material significantly [10] [16]. This research contributes significantly to the use of modern and innovative materials to obtain low-density concrete while maintaining the mechanical characteristics as well as improve flexural strength if it is utilized in concrete slabs and overcome the problems of rust of main steel reinforcement. By combining some of the previous studies, we can summarize them as follows:
Fiber-reinforced polymer composites (FRPCs) have developed as a relatively new construction material capable of addressing many of the shortcomings of existing construction materials [17]. Glass Fibre Reinforced Polymer (GFRP) bars are a non-corrosive alternative reinforcing solution for concrete constructions that are less expensive to maintain and repair [18]. This non-magnetic alternative reinforcement is lighter than steel and has a higher tensile strength [19], [20]. GFRP bars have been effectively utilized as internal reinforcement for concrete structures in many studies, including beams [21], columns [22], and slabs [23]. Because slabs are the main structural parts in a structure, they consume the most concrete and contribute significantly to the dead load [24]. They should be constructed to use the least amount of resources and to be as light as possible. Previous studies [25], [26], drilled holes in the slab to lower the amount of concrete utilized and the overall weight.
Polymers improve the mechanical and chemical characteristics of concrete, including increased compressive strength, flexural and tensile strength, good performance and durability, and reduced corrosion and permeability. Polymer impregnated concrete (PIC), polymer concrete (PC), and polymer-modified concrete are the three types of concrete that contain polymer (PMC). Polymer concrete has higher compressive, tensile, and flexural strengths, durability, low permeability, reduced corrosion, excellent adhesion to concrete and steel, and resistance to freezing, thawing, and acid attacks. However, the disadvantages include higher costs, more complex runs, and contractor unfamiliarity. Polymer concrete is appropriate for marine works, nuclear power plants, sewage works, desalination plants, corrosion-prone pipes and pumps, tunnel lining, and storage water tanks.
Victor Y. Garas et al., (2003), Polymer Concrete (PC) composites have a different set of characteristics that vary depending on the formulation. The variations in polyester polymer concrete mixture components that affected the characteristics were examined in this study. The impact of resin content, aggregates, fibers, and coupling agents was scrutinized. It was discovered that the optimum polymer content ranged from 12% to 14% (w/w). Utilizing fibers and coupling agents improved the mechanical characteristics of PC even more. In addition, a new database was created to document various PC characteristics [27].
Jiang Cong-sheng et al. (2004) The influence of polymer addition on the microstructure, performance, and mechanical characteristics of lightweight aggregate concrete was looked at. The addition of polymer to lightweight aggregate concrete increased its performance and mechanical qualities. The improvement of mechanical characteristics was determined to be due to the alteration of microstructural homogeneity and densification with the addition of polymer. The lightweight aggregate concrete with 13 percent ethylene-acetate ethylene inter-polymer (EVA) demonstrates favorable mechanical qualities in compressive strength and bending strength [28].
Gouda et al. (2009) discussed the most parameters that might affect the behavior of the GFRP reinforced columns. This included replacing main longitudinal steel and stirrups with GFRP bars and sheets in two forms. Those forms were warped the longitudinal reinforcement of the column and bent the square column from the outside. Also, the reinforcement percentage was taken as a variable [29].
Tejas Joshi et al. (2016) The impact of different aggregate sizes and water-cement ratios on pervious concrete was investigated. The experiments are carried out to determine the void ratio, permeability, strength, and density of the material. It was discovered that when the strength increased, the density began to rise as well [30].
Yehia et al. (2017) Porous concrete's characteristics were investigated to expand its structural engineering applications. Physical parameters including density, permeability, and porosity, as well as mechanical parameters like compressive strength, indirect tensile strength, and flexural strength, were investigated. Increased cement content increases compressive stength, indirect tensile strength, and flexural strength, according to the findings. Porous concrete has a lower density than conventional concrete by 21%, yet its permeability factor is sixteen times that of normal concrete. Although increasing the cement content has no impact on the ultimate load or maximum deflection of polymer impregnated porous concrete slabs, the results demonstrate that this concrete can be utilized in structural engineering applications and that it is simple to cast special concrete-like polymer concrete without the use of special tools [31].
Yehia et al., (2020), The research of fresh and hardened concrete characteristics was presented in this research. To get the best ratio, eighteen samples were made with three different mixing ratios (samples mostly consist of cement, water, and polyester) as fixed proportions, but the total coarse weight as a variable factor. In addition to taking into account the concrete density and void ratio, the collapse mechanism was observed and categorized. The researcher concluded from the experimental data that increasing the total coarse weight in the fine-free mixture increases the density of the samples while also increasing their flexural strength, compressive strength, and indirect tensile strength [32], [33].
-
EXPERIMENTAL WORK PROGRAM:
Porous lightweight concrete was produced by utilizing two types of aggregate; Lightweight Expanded Clay Aggregate (LECA) with replacement ratio (0, 15, and 25 %) and crushed stone aggregate (dolomite). Two different cement contents were utilized (200kg/m3 and 350 kg/m3), and resin (unsaturated polyester in addition to peroxide as catalyze) with ratio (0 and 15%) from concrete volume were utilized to study its integrity with GFRP bars on the results. Compressive strength, indirect tensile strength, flexural strength, and dry density of different concrete mixtures were examined after they had hardened. Study of the structural behavior of polymer impregnated porous concrete slabs in bending. Table (1) demonstrates the different mixes studied in this research. Mix (1) contains no LECA, while mix (2) has less dolomite than a mix (1) in addition to the presence of LECA, Mix (3) contains more LECA and less dolomite than a mix (2). Regarding mix (4), it is similar to mix (1) but has different cement content and more water content. Mix (5) is similar to mix (2) but with other cement content and water content. The same case can be seen in comparing mix (3) and mix (6).
Concrete ingredients were tested according to ES:1109/2002 [34] for aggregate and ES: 4756-1/2009 [35] for cement. Table (2) demonstrates the physical characteristics of the utilized crushed stone, table (3) demonstrates the sieve analysis results for coarse aggregate, table (4) demonstrates the physical characteristics of the utilized sand, table (5) demonstrates the characteristics of utilized cement (CEM I 42.5N), table (6) demonstrates the physical characteristics of the LECA aggregate, table (7) demonstrates the sieve analysis results for LECA aggregate and table (8) demonstrates the physical characteristics of the utilized unsaturated polyester, also table (9) demonstrates the characteristics of GFRP bars. It's worth to be mention that clean tap drinking water was utilized.
Table (1): Mix Design Quantities for different Mixes
Mix No.
Coarse Aggregate (Kg/m3)
Cement (Kg/m3)
Water (Liter)
w/c
Admixture
Dolomite
LECA
M-1
1500
0
200
60
0.3
1% of Cement Content (HRWR)
M-2
1275
225
200
60
0.3
M-3
1125
375
200
60
0.3
M-4
1500
0
350
105
0.3
M-5
1275
225
350
105
0.3
M-6
1125
375
350
105
0.3
Table (2): The physical characteristics of the crushed stone that was utilized
Test
Results
Acceptable limit
Specific Gravity
2.67
–
Unity Weight (t/m3)
1.57
–
Materials Fine than No. 200 Sieve
1.85
Less than 3%
Absorption %
1.95
Less than 2.5%
Abrasion
16.85
Less than 30 %
Crushing magnitude
20.00
Less than 30 %
Impact
12.85
Less than 45%
Table (3): Sieve Analysis Results for Coarse Aggregate
Sieve Size (mm)
37.5
19
9.5
4.75
2.36
Pan
% Passing
100
96.2
60.8
0.9
0.1
0
Table (4): The Physical Characteristics of the Utilized Sand
Test
Results
Acceptable Limit
Specific Gravity
2.65
–
Unit Weight (t/m3)
1.75
–
Materials Finer than No 200 Sieve
1.50
3%
Absorption %
1.05
2.5%
Table (5): Characteristics of Utilized Cement (CEM I 42.5N)
Characteristics
Measured Magnitudes
Limits of the E.S.S*
Fineness (cm2/gm)
3460
–
S. G.
3.15
–
Expansion (mm)
1.30
Not more than 10
The consistency of Standard Cement Paste (Water Percentage)
26%
–
I. S. T. (min)
155
Not less than 60 min
F. S. T. (min)
200
–
Compressive Strength (N/mm2)
2 days
21.7
No less than 10
7 days
48.6
–
28 days
59.4
No less than 42.5 and not more than 62.5
Chemical Compositions
SiO2
21.16%
–
Al2O3
4.98%
–
Fe2O3
3.78%
–
CaO
64.39%
–
MgO
1.36%
–
SO3
1.95%
–
Loss Ignition %
1.35%
–
*Egyptian Standard Specifications No:4756-1/2009[19].
Table (6): Physical Characteristics of the LECA Aggregate
Property
Obtained Results
Specific Gravity
0.49
Unit Weight (t/m3)
1.08
Table (7): Sieve Analysis Results for LECA Aggregate
Sieve Size (mm)
19
16
11.2
8
5.6
Pan
% Passing
100
89.6
29.9
1.5
0.9
0
Table (8): Physical Characteristics of the Unsaturated Polyester Utilized
Characteristics
Obtained* Magnitudes
Density (g/cm3)
1.09
E (N/mm2)
3.3×103
F. Str. (N/mm2)
45
Ten. Str. (N/mm2)
40
Max. Elongation (%)
1
Visc. at 25o C (cP)
250
* Data was obtained from the source.
Table (9): Characteristics of GFRP Bars
Characteristics
Obtained* Magnitudes
Volume Fraction (%)
60%
Actual Diameter (mm)
11.8
Tensile Strength (kg/cm2)
4750
An experimental work program consisting of two different phases was prepared. Phase 1 included testing of cubes (10x10x10) cm in determining compressive strength at 7 and 28 days as demonstrated in fig. (1), testing of cylinders (10 x 20) cm to determine indirect tensile strength at 28 days as demonstrated in fig.(2) and finally, testing prisms (10x10x50) cm to determine flexural strength 28 days as demonstrated in fig.(3). Phase two included testing six slabs with dimension (10x50x120) cm and reinforced with (GFRP) mesh with a diameter of 12mm. Only three slabs were impregnated with 15% resin of slab volume and were tested under the impact of bending moment. The test setup of slabs can be seen in fig. (4) with two hinged ends and one concentrated load in the middle.
Fig. (1): Test of compressive strength Fig. (2): Test of Indirect tensile strength Fig. (3):Test of Flexural strength
-
RESULTS, ANALYSIS, AND DISCUSSIONS:
A digital testing machine with a capacity of 200 tons was utilized. The testing was carried out per BS:12390 [36]. Cubes, cylinders, and prisms were tested for compressive strength, indirect tensile strength, and flexural strength after (7) and
(28) days, respectively. The test results for all mixtures were summarized in table (10) below. It was also discovered that steel fibers improved the mechanical characteristics of both traditional and lightweight concrete at various ages. The improvement in traditional concrete was more noticeable than in lightweight concrete. The reason for this can be attributed to the percentage of aggregate utilized in conventional concrete. Furthermore, the use of GFRP external sheet increased the enhancement magnitude. The hardened property test results for all mixes are demonstrated in the table (10) below.
Table (10): Characteristics of Tested Specimens
The Tested Property
Mix Name
M-1
M-2
M-3
M-4
M-5
M-6
*
**
*
**
*
**
*
**
*
*
Density(t/m³)
1.52
2.41
1.47
2.33
1.44
2.28
1.66
2.16
1.61
1.57
7-days compressive strength (kg/cm2)
10.8
–
23
–
39
–
42.6
–
49.3
43.6
28 days Compressive strength (kg/cm2)
15
60
30
69
48
57
60
90
65
56
Indirect tensile Strength (kg/cm2)
5
8
6.2
9.2
6.35
9.3
11
–
12
10
Flexural strength (kg/cm2)
9
41
15.4
37.2
18
27
19
45
18.2
20
*as an Average of Three Repeats.
7
6.5
6
6
5.6
M1
M2
M3
M4
M5
M6
Mix
5
4.8
4.93
4.26
4.36
4
3.9
3
3
2.3
7 Days
28 Days
2
1.5
1.08
1
0
4
3
3
2
Compressive Strength (Mpa)
Compressive Strength (Mpa)
Fig. (4): Compressive strength of various mixes after 7 and 28 days
7
6.5
6
6
5.6
5
4.8
1
0
0
0
M1
M4
M2
M5
M3
M6
Mix Name
1.5
Fig. (5): Compressive strength for different mixes
7
6.5
6
6
5.6
5
4.8
1
0
0
0
M1
M4
M2
M5
M3
M6
Mix Name
4
3
3
2
1.5
1.82
1.8
1.54
1.5
1
0.9
Indirect Tensile Strength (Mpa)
Flexural Strength (Mpa)
Fig. (6): Indirect tensile strength for different mixes
2.5
2
1.9
2
0
0
0
M1
M4
M2
M5
M3
M6
Mix Name
0.5
Fig. (7): Flexural strength for different mixes
1700
1662.5
1650
1614
1600
1572
1550
1520
1400
1350
1300
M1
M2
M3
M4
M5
M6
Mix Name
1500
1472.5
1450
1435
6.5
6
6
5.7
5.6
4.8
3
Density (Kg/cm3)
Compressive Strength (MPa)
Fig. (8): Density for different mixes various LECA replacement
8
7
6
5
4
3
2
1
0
6.9
M1 M2 M3 M1+ M2+ M3+ M4 M5 M6
Polyester Polyester Polyester
Mix Name
1.5
Fig. (9): Various LECA replacement and their impact on compressive strength for different mixes
Flexural Strength (MPa)
Fig. (10): Various LECA replacement and its impact on indirect tensile strength for different mixes
4.5
4
3.5
3
2.5
2
1.5
1
0.5
0
M1
M2
M3
M1+ M2+ M3+
M4
M5
M6
Polyester Polyester Polyester
Mix Name
Fig. (11): Various LECA replacement and their impact on flexural strength for different mixes
10
9
8
7
6
5
4
3
2
1
0
M1
M1
M2
M2
M3
M3
M4
M4
Mix Name
Compressive Strength (MPa)
Fig. (12): The impact of polyester content on compressive strength for various mixtures.
Fig. (13): The impact of polyester content on indirect tensile strength for various mixtures.
p>Fig. (14): The impact of polyester content on flexural strength for different mixes various mixtures.
Fig. (4) demonstrates the mix name and the corresponding compressive strength at 7days and 28days. The fig. indicates that the compressive strength at 28 days is higher than at 7and 28 days by 71% – 80%. Fig. (5) demonstrates the impact of cement content on compressive strength for all different mixes. The test results demonstrate that by increasing the cement content from 200 Kg/m3 to 350 Kg/m3, the compressive strength at age seven days increased by 300%. This can result from the comparison between mix (1) and (4). The impact of increasing the cement content on the compressive strength at age 7 and 28 days decreases as the percentage of LECA increases. Compressive strength at 28 days increased by two times, as seen from the comparison between mix (3) and (6). When mix (1), (2), and (3) are compared, the impact of LECA replacement is discussed. As can be seen in the figure, LECA replacement increases compressive strength by up to 2 times. The cement content raised the indirect tensile strength by 20%, as demonstrated from fig. (6). From the comparison of mix (1), (2), and (3), LECA replacement increases indirect tensile strength by up to 27%. Fig. (7) demonstrates the flexural strength of different mixes, where the cement content can improve the results by 11% when increased according to the comparison between mix (2) and (5). The impact of increasing LECA is about 100% depending on the comparison between mix (1), (2), and (3). The density of different mixes is demonstrated in fig. (8), where changing the percentages of LECA has no significant impact on the results. Fig. (9) reveals the impact of LECA and polyester on the compressive strength; compressive strength decreased by 6% when polyester was added to the mix that contained LECA with 15% replacement. On the contrary, indirect tensile strength is improved by 30% when the polyester was added with LECA with 15% replacement, as demonstrated in fig. (10). No clear impact to the results was noticed when adding polyester to LECA with 25% replacement. The flexural strength was slightly decreased by 4% when 15% polyester was added to LECA with 15% replacement, according to fig. (11). Adding only the polyester to the mix by 15% increased the compressive strength by 300%, as demonstrated in fig. (12). In comparison, it increased by only 66% in the presence of LECA. The impact of polyester was 66% improvement in the indirect tensile strength, but it was 355% in the presence of LECA, as illustrated in fig. (13). Flexural strength of mix (1) increased by 3.5 times when polyester was added, but it increased by 36% in the presence of LECA and polyester, as demonstrated in fig. (14).
The load-deflection curve based on test results of slabs is discussed in fig. (15). It demonstrates brittle behavior for S1 and S6, meanwhile ductile behavior is observed for S4 and S5. S4 is more ductile than S1 because of the increased cement content. The presence of LECA decreased the ductility of specimens, which is clear from the comparison between S4, S5, and S6. Surprisingly, the opposite happened for S1, S2, and S3. It is obvious that S3 is more ductile than S2. The deflection of S1 was 33 mm. Meanwhile, it was 15 mm for S6. On the other hand, the deflection of S4 was 25 mm.
S1 S2 S3 S4 S5 S6
35
30
25
Load (ton)
20
15
10
5
0
-5 0 5 10 15 20 25 30 35
-5 Deflection (mm)
Fig.(15): Load deflection curve of slabs
Load (ton)
From fig. (16) and (17), it is concluded that slab S3 had less mid-span deflection and ductility by 33.2% and 45%, respectively than S2. For slabs with 350 kg/m³ cement content utilizing 25% LECA replacement, there was an increase in mid- span deflection, reduction in stiffness, and decrease in ultimate load by 65% than 15% LECA replacement. For slabs that contained 15% LECA replacement, 200 kg/m³ cement content and impregnated with 15% polyester cracking load, failure load and ultimate mid-span deflection increased by 64%, 141%, and 60% respectively than slab containing 350kg/m³ cement content without polyester. Utilizing 25% LECA replacement and 350 kg/m³ cement content gave a similar slab behavior as utilizing 0% LECA replacement and 200 kg/m³ cement content.
S2 CONC. STRAIN
S3 conc
S1 REINF. STRAIN
S3 REINF 30
25
20
15
10
5
0
S2 REINF STRAIN
0 0.002 0.004 0.006 0.008 0.01 0.012 0.014
Strain
Fig.(16) Load and strain curve of slabsS1, S2, and S3
S4 CONC STRAIN
S5 REINF. STRAIN
S4 REINF STRAIN
S6 FIBER STRAIN
S5 CONC. STRAIN
35
30
25
20
15
10
5
0
0
0.005
0.01
Strain
0.015
0.02
Load (ton)
Fig.(17) Load and strain curve of slabs S4, S5, and S6
-
CONCLUSIONS:
The compressive strength in seven days is 71 percent to 80 percent of the compressive strength in 28 days, according to the experimental results. In 0 percent LECA replacement mixtures, increasing the cement content from 200 to 350 kg / m3 increases the compressive strength, indirect tensile strength, and bending strength by 300 percent, 120 percent, and 111.1 percent, respectively, while LECA replacement at 15% and 25% decreases the density by 3.1 percent and 5.6 percent, respectively. Compressive strength increased by 100 percent and 220 percent, indirect tensile strength increased by 24 percent and 27 percent, and flexural strength increased by 71 percent and 100 percent for mixtures containing 200 kg/m3 of cement and 0 percent LECA substitution, for mixtures containing 200 kg/m3 of cement and 0 percent LECA substitution, for mixtures containing 200 kg/m3 of cement and 0 percent LECA substitution, for mixtures containing 200 kg/m3 of cement and
It has also been discovered that utilizing 25% LECA substitute and 350 kg/m3 cement content produces the same slab behavior as utilizing 0% LECA substitute and 200 kg/m3 cement content. As a site approach, utilizing slurry cover as a shutter for impregnated polyester would be highly cost-effective; however, for testing purposes, it leads to a lot of measurement inaccuracies.
REFERENCES:
[1] Z. S. Al-Khafaji and M. W. Falah, Applications of high density concrete in preventing the impact of radiation on human health, J Adv Res Dyn Control Syst, vol. 12, no. 1 Special Issue, 2020, doi: 10.5373/JARDCS/V12SP1/20201115. [2] Z. S. Al-Khafaji et al., The Impact of Using Different Ratios of Latex Rubber on the Characteristics of Mortars Made with GGBS and Portland Cement, in IOP Conference Series: Materials Science and Engineering, 2021, vol. 1090, no. 1, p. 12043. [3] M. W. Falah, A. A. Hafedh, S. A. Hussein, Z. S. Al-Khafaji, A. A. Shubbar, and M. S. Nasr, The Combined Effect of CKD and Silica Fume on the Mechanical and Durability Performance of Cement Mortar, in Key Engineering Materials, 2021, vol. 895, pp. 5967. [4] Z. S. Al-Khafaji et al., The Impact of Using Different Ratios of Latex Rubber on the Characteristics of Mortars Made with GGBS and Portland Cement, IOP Conf Ser Mater Sci Eng, vol. 1090, no. 1, p. 012043, 2021, doi: 10.1088/1757-899x/1090/1/012043. [5] A. A. Shubbar, Z. S. Al-khafaji, M. S. Nasr, and M. W. Falah, USING NON-DESTRUCTIVE TESTS FOR EVALUATING FLYOVER FOOTBRIDGE: CASE STUDY. [6] M. W. Falah, Y. A. Ali, M. Z. Al-Mulali, and Z. S. Al-Khafaji, Finite Element Analysis Of CFRP Effects On Hollow Reactive Powder Concrete Column Failure Under Different Loading Eccentricity, Solid State Technol, vol. 63, no. 2, 2020. [7] A. L. I. SHUBBAR, Z. Al-khafaji, M. Nasr, and M. Falah, Using non-destructive tests for evaluating flyover footbridge: case study, Knowledge- Based Eng Sci, vol. 1, no. 01, pp. 2339, 2020. [8] G. Zhang et al., Reinforced concrete deep beam shear strength capacity modelling using an integrative bio-inspiredalgorithm with an artificial intelligence model, Eng Comput, pp. 114, 2020. [9] H. Tao et al., Artificial intelligence models for suspended river sediment prediction: state-of-the art, modeling framework appraisal, and proposed future research directions, Eng Appl Comput Fluid Mech, vol. 15, no. 1, pp. 15851612, 2021. [10] D. S. Hanoon et al., Early age assessment of cement mortar incorporated high volume fly ash, in IOP Conference Series: Materials Science and Engineering, 2021, vol. 1090, no. 1, p. 12019. [11] A. A. Shubbar, M. Sadique, M. S. Nasr, Z. S. Al-Khafaji, and K. S. Hashim, The impact of grinding time on properties of cement mortar incorporated high volume waste paper sludge ash, Karbala Int J Mod Sci, vol. 6, no. 4, 2020. [12] D. N. Jabbar, A. Al-Rifaie, A. M. Hussein, A. A. Shubbar, M. S. Nasr, and Z. S. Al-Khafaji, Shear behaviour of reinforced concrete beams with small web openings, Mater Today Proc, 2021. [13] A. A. Shubbar et al., Properties of cement mortar incorporated high volume fraction of GGBFS and CKD from 1 day to 550 days, J Build Eng, vol.30, p. 101327, Jul. 2020, doi: 10.1016/j.jobe.2020.101327.
[14] S. F. Al-Mamoori et al., Production of Ternary Blend Binder as an Alternative to Portland Cement, in IOP Conference Series: Materials Science and Engineering, 2021, vol. 1090, no. 1, p. 12069. [15] A. Shubbar, M. Nasr, M. Falah, and Z. Al-Khafaji, Towards net zero carbon economy: Improving the sustainability of existing industrialinfrastructures in the UK, Energies, vol. 14, no. 18, p. 5896, 2021.
[16] H. M. Al-Baghdadi, A. A. F. Shubbar, and Z. S. Al-Khafaji, The Impact of Rice Husks Ash on Some Mechanical Features of Reactive Powder Concrete with High Sulfate Content in Fine Aggregate, Int Rev Civ Eng, vol. 12, no. 4, pp. 248254, 2021. [17] W. Ferdous, T. D. Ngo, K. T. Q. Nguyen, A. Ghazlan, P. Mendis, and A. Manalo, Effect of fire-retardant ceram powder on the properties of phenolic-based GFRP composites, Compos Part B Eng, vol. 155, pp. 414424, 2018. [18] H. A. Abdalla, Evaluation of deflection in concrete members reinforced with fibre reinforced polymer (FRP) bars, Compos Struct, vol. 56, no. 1, pp.6371, 2002.
[19] W. Ferdous, A. Manalo, and T. Aravinthan, Effect of beam orientation on the static behaviour of phenolic core sandwich composites with different shear span-to-depth ratios, Compos Struct, vol. 168, pp. 292304, 2017. [20] A. C. Manalo, Behaviour of fibre composite sandwich structures under short and asymmetrical beam shear tests, Compos Struct, vol. 99, pp. 339 349, 2013. [21] G. B. Maranan, A. C. Manalo, B. Benmokrane, W. Karunasena, and P. Mendis, Evaluation of the flexural strength and serviceability of geopolymer concrete beams reinforced with glass-fibre-reinforced polymer (GFRP) bars, Eng Struct, vol. 101, pp. 529541, 2015. [22] G. B. Maranan, A. C. Manalo, B. Benmokrane, W. Karunasena, and P. Mendis, Behavior of concentrically loaded geopolymer-concrete circular columns reinforced longitudinally and transversely with GFRP bars, Eng Struct, vol. 117, pp. 422436, 2016. [23] K. Bouguerra, E. A. Ahmed, S. El-Gamal, and B. Benmokrane, Testing of full-scale concrete bridge deck slabs reinforced with fiber-reinforced polymer (FRP) bars, Constr Build Mater, vol. 25, no. 10, pp. 39563965, 2011. [24] R. Sacks, C. M. Eastman, and G. Lee, Parametric 3D modeling in building construction with examples from precast concrete, Autom Constr, vol.13, no. 3, pp. 291312, 2004.
[25] V. C. de Castilho, M. do Carmo Nicoletti, and M. K. El Debs, An investigation of the use of three selection-based genetic algorithm families when minimizing the production cost of hollow core slabs, Comput Methods Appl Mech Eng, vol. 194, no. 4547, pp. 46514667, 2005. [26] M. Pajari, Web shear failure in prestressed hollow core slabs, J Struct Eng, vol. 42, no. 4, pp. 83104, 2009. [27] V. Y. Garas and C. Vipulanandan, Review of polyester polymer concrete properties, Rep Innov Grouting Mater Technol (CIGMAT), Houston, TX, 2003. [28] J. Cong-sheng et al., Influence of polymer addition on performance and mechanical properties of lightweight aggregate concrete, Wuhan Univ J Nat Sci, vol. 9, no. 3, pp. 348352, 2004. [29] Y. Sameh, Behavior of short RC columns reinforced with FRP bars, – , no. 2 (12), pp. 152161, 2009. [30] T. Joshi and U. Dave, Evaluation of strength, permeability and void ratio of pervious concrete with changing W/C ratio and aggregate size, Int J Civ Eng Technol, vol. 7, no. 4, pp. 276284, 2016. [31] S. Yehia and M. M. Fawzy, The impact of using Polymer Impregnated Porous Concrete in Structural Engineering Applications, Int J Curr Eng Technol Inpressco, vol. 7, no. 2, 2017. [32] S. Yehia and M. W. Falah, Study on Fresh and Hardened Characteristics of Polymer Impregnated No-Fines Concrete, Artic Int J Adv Sci Technol, vol. 29, no. 3, pp. 1347213483, 2020, [Online]. Available: https://www.researchgate.net/publication/344192846. [33] Q. S. R. Marshdi, S. A. Hussien, B. M. Mareai, Z. S. Al-Khafaji, and A. A. Shubbar, Applying of No-fines concretes as a porous concrete in different construction application, Period Eng Nat Sci, vol. 9, no. 4, pp. 9991012, 2021. [34] E. S. Specifications, ES 1109/2002: Concrete aggregates from natural sources, Egypt Organ Stand Qual Cairo, Egypt, 2002. [35] E. S. M. Committee, Cement Part1: Composition, specifications and conformity criteria for common cements; ES 4756-1. Cairo (Egypt): Egyptian Organization for Standards and Quality, Eos (Washington DC), 2009. [36] B. Standard, Testing hardened concrete, Compressive Strength Test Specimens, BS EN, pp. 1239012393, 2009.