
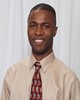
- Open Access
- Authors : Etim S Udoetok , Tryfon T Charalampopoulos
- Paper ID : IJERTV10IS050009
- Volume & Issue : Volume 10, Issue 05 (May 2021)
- Published (First Online): 06-05-2021
- ISSN (Online) : 2278-0181
- Publisher Name : IJERT
- License:
This work is licensed under a Creative Commons Attribution 4.0 International License
Stoichiometry Effects in Proton Exchange Membrane Fuel Cells
Etim S Udoetok, Tryfon T Charalampopoulos
Mechanical Engineering Department Louisiana State University
Baton Rouge, LA-USA
Abstract In this paper, a study of the effects of stoichiometry on the performance of proton exchange membrane fuel cells is presented. Theoretical analysis and experiments were used to show that the electrodes area ratio has effects on stoichiometry of fuel cell reactions and consequently on the cell voltage and on the efficiency of a proton exchange membrane fuel cell. Experimental data was used to estimate the effect of equal electrodes area on the fuel cell reaction. The active electrodes area ratios were adjusted to enhance stoichiometry and consequently improve performance, but improvement was limited due to the low thickness of the membrane. Efficiency values obtained match values published by others. The study concluded that the equal areas electrode-membrane sandwich design of proton exchange membrane fuel cells affects the stoichiometry of the fuel cell reaction and consequently results in efficiency lower than that of the stoichiometric reaction.
Keywords Fuel cell, PEM and electrode
-
INTRODUCTION
A fuel cell can be defined as an electrochemical device used to convert the chemical energy of a fuel into direct current electrical energy [1]. A fuel cell works by combining a fuel and oxidizer in an anode half-cell reaction and a cathode half-cell reaction. These half-cell reactions unify to form a controlled combustion reaction. Therefore, fuel cells produce electricity like conventional batteries, but unlike conventional batteries, fuel cells run on fuels and oxidizers and are theoretically expected to work continuously as long as fuel and oxidizer are supplied [1-4]. Thermodynamically, fuel cells offer efficiencies in the range of 80 to 90%, but practical fuel cell efficiency are usually lower [1, 2]. Fuel cells potential attracted a bump in research in the 2000 [5, 6], but the progress has been slow over the years.
The development of fuel cells whose electrolytes are membranes that allow proton transport by Grubb and Niedrich
[7] at General Electric boosted the interest in fuel cells technology, since Proton-Exchange Membrane (PEM) fuel cells operate at low temperatures and pressures, and has no electrolyte leakage problem. Dow Chemicals development of a new perfluoro-sulfonic acid proton exchange membrane with the trade name Nafion pushed PEM fuel cell technology beyond space application to automobile, medical, domestic, industrial and other applications involving electric power [8- 10]. Immediate needs for PEM fuel cells included low cost materials, high proton conductivity membrane, porous electrodes, durable materials, etc. [11-14]. High efficiency is not the main advantage of PEM fuel cells [1]; however, efficiency is important in understanding and analyzing theperformance of fuel cells [15], and getting the analysis correct is crucial to advancing PEM fuel cells. Thermodynamically, it is important to understand that the source of energy is the chemical energy (H) released by the controlled combustion of the fuel supplied and the work output is the electrical energy (~G) generated, while the rest of the energy component from the source in the form of direct heat and other minor losses are lost to the environment. Works on developing fuel cells includes optimizing flow field designs [16], direct application of electrodes to membrane [17], finding best operating conditions [18, 19] and more, but fuel cells still performing lower than expected practically; therefore, there is more room for developing fuel cells. Some authors have attributed the minor losses to activation, concentration, ohmic and other factors in order to account for the practical performance of fuel cells [20-22].
In this paper, work on the effects of electrodes area ratio on the stoichiometry of PEM fuel cell reactions and its effect on the performance of PEM fuel cell is presented.
-
METHODS
In normal fuel cells, the electrochemical half reactions occur at the electrodes. The half reaction at the anode starts slowly and independently by use of catalysts and potential across the electrolyte, but thereafter, the anode electrode reaction connects to the cathode electrode reaction and the reaction rate goes up and steadies. The reaction layers at the electrodes surfaces are very thin and have not yet been measured practically [3]. The electrochemical reaction occurs on the surface area of the electrodes, so increasing the surface area increases the reaction rate [3]. For high reaction rate and consequently high current density, electrode reactive areas are usually increased by increasing porosity. If increase in electrode area results in higher power, then it is possible that the electrodes area ratio has effect on the stoichiometry of the cell reaction. In this section, the effect of the equal electrode areas will be studied analytically. Proton exchange membrane fuel cells, alkaline fuel cells and phosphoric acid fuel cells are usually fabricated with electrodes, which have equal area and equal thickness. It is usually a sandwich-like design.
Fuel cells overall reactions are controlled combustion reactions, so it is desired that the fuel cell reaction be at least stoichiometric in order to unleash a high amount of the chemical energy stored in the fuel. Calculation results for cases A and B presented in Table 1 were obtained assuming stoichiometric fuel cell reaction and assuming other losses are negligible. Case A is PEM fuel cell operation with O2 as
2
2
oxidizer, while case B is for PEM fuel cell operation with air as oxidizer. The effects of the equal area electrodes are ignored,
(1Area) H 2 ( 1 Area)O2 (1Area)H 2 O
and the free energy change, generated voltage, and efficiency
Anode Cathode
are evaluated for each case. The stoichiometric condition equations for operation with oxygen and air are given as equations (1) and (2) below
( A)H 2 (0.5A)O2 ( A)H 2 O
and for H2-air PEM fuel cell operation
(4)
H 2 1 O2 H 2 O
(1)
Anode
Cathode
2
H 1 O 1.88N H O 1.88N
(A)H 2
(0.5A)O2 (1.88A)N 2
(A)H
2O 1.88N 2
2 2 2 2 2 2
(2)
(5)
The calculation results indicate that the cell produces the same electrical energy (G) and voltage and have the same efficiencies. Practically, different results are obtained because of the effect of equal electrode areas. The effect of the electrode area does not only make the output of H2-O2 PEM fuel cell and H2-Air PEM fuel cell different, it also affects the practical efficiency of the fuel cells.
Table 1: Calculation results for PEM fuel cells assuming stoichiometric reactions and operating under the conditions of
Case
A
B
Oxidizer
Oxygen
Air
Overall Cell Reaction
H 2 1 O2 H 2 O
2
H 2 1 O2 1.88N 2
2
H 2O 1.88N 2
Enthalpy,
H (kJ/kmol)
-241845
-241845
Entropy,
S (kJ/K.kmol)
-13.231
-13.231
Free Energy Change,
G (kJ/kmol)
-228613.8
-228613.8
Cell Voltage, E (V)
1.185
1.185
Efficency, (%)
94.52
94.52
Case
A
B
Oxidizer
Oxygen
Air
Overall Cell Reaction
H 2 1 O2 H 2 O
2
H 2 1 O2 1.88N 2
2
H 2O 1.88N 2
Enthalpy,
H (kJ/kmol)
-241845
-241845
Entropy,
S (kJ/K.kmol)
-13.231
-13.231
Free Energy Change,
G (kJ/kmol)
-228613.8
-228613.8
Cell Voltage, E (V)
1.185
1.185
Efficiency, (%)
94.52
94.52
298K and 1 atm
The electrodes are assumed to have the same porosity and thickness, so area, A, represents the area of the electrode. The area being considered in this case is similar to the area used for calculating current density. It is the area that is usually specified on commercial electrodes or in the specifications of a new fuel cell e.g. a 25 cm2 electrode. On the left hand side of Equation (4), the coefficient of H2, which is A, represents the area of the anode, while the coefficients of O2, which is ½A, represents the cathode area. In other words, the active area of the cathode must be half the area of the anode for the cell reaction to be stoichiometric. Similarly, on the left hand side of equation (5), the coefficient of H2, which is A, represents the area of the anode, while the sum of the coefficients of O2 and N2, which is 2.28A, represents the cathode area. In other words, the active area of the cathode must be 2.88 times the area of the anode for the cell reaction to be stoichiometric. The analysis used to obtain equations (4) and (5) reveals that equations (1) and (2) do not represent the reactions that occur in fuel cells with equal area electrodes.
In order to analyze the actual performance of PEM fuel cells by considering the effects of the equal area electrodes, the free energy change, generated voltage and efficiency are evaluated
for both the cases of operation of PEM fuel cell with O2 as
oxidizer and with air as oxidizer. The cell reaction equations are re-written to reflect the effects of the equal area electrodes as shown in equations (6) and (7) below
The reaction equations, equations (1) and (2), are valid only for system where there is actual mixing of the reaction species such as in a combustion chamber. The stoichiometric volume
H 2 O2
H
2 O2
1 H
O 1 O
2 2 2
(6)
ratio of reactants is usually found as shown below:
H 0.21O 0.79N H O 0.79 H 0.21 H O 0.79N
2 2 2 2 2 2 2 2 2 2
2
2
H 2 (g) 1 O2
-
H
2 O(g)
(7)
2
2
(1 mol) H 2 ( 1 mol)O2 (1mol)H 2 O
2
2
(V) H 2 ( 1 V )O2 (V )H 2O
(3)
The intermediate reactions between the reactants are connected by the electrode surface areas, so the volume ratio of the products is not considered in the analysis. The operation of
Setting the ratio of reactants volumetric flow rates in order to match stoichiometric reaction has negligible positive effect because the reactants are separated by the electrodes. According to some authors, the absorption rate of the reactants at the electrode surface are limited and even adjusting pressures at the electrode has limited positive effect [23, 24], since the absorption of the reactants are driven by the separate electrode reaction rates rather than a free pass. Balancing the volumetric flow rates to achieve stoichiometry would also cause uneven pressure across the cell. Since on the electrodes, the reaction layer thickness tends to zero, it is safe to assume that the thickness of the reaction layer at the anode equals that at the cathode. Now, having assumed equal reaction layers at the electrode surface areas, equation (3) can be modified as shown below:
equal electrodes area PEM fuel cell results in the formation of H2O2 due to the electrolytic nature of the reaction and more H2O2 is formed when O2 is used as an oxidizer due to the excess O2. The formation of H2O2 has negative impact on the membrane [25] and on the fuel cell performance. However, most terrestrial fuel cell application involves the operation of fuel cells with air as oxidizer [4], so more attention has been paid to the analysis of PEM fuel cell operation with air as the oxidizer.
The hydrogen on the right hand side of equation (7) is left there because it is formed on the cathode side, which is also the exhaust side. The formation of H2 is a negative effect, since it leads to the loss of fuel and energy to the environment. The first effect of using equal electrodes areas can be seen in equations (6) and (7), which are not stoichiometric, as the
appearance of H2 and H2O2 in the products. and are to be found from experimental data.
-
-
EXPERIMENTS
PEM Fuel cells with conventional equal electrode areas were setup and ran for operations with O2 and air. Then the fuel cells electrodes area ratios were adjusted for stoichiometry by using polyethylene leather to cover part of the electrode areas (see Fig. 1[26]). The experiments were
Fig. 1: PEM fuel cell electrode partially covered with polyethylene sheet.
run at the same flow rate for comparison. The fuel cells were loaded, readings were recorded, and the recorded values were used to obtain plots in Figs. 2-5 [26]. The same anode area was used to ensure that cell is exposed to same amount of fuel for reasons of comparison. I.e. all the H2-O2 PEM fuel cell experiments were performed using a fully exposed anode area (1A) while all the H2-Air PEM fuel cell experiments were performed using a partly exposed anode area (0.42A).
Fig. 2: Plot of voltage vs. current for H2-O2 PEM FC electrodes area ratio experiment.
Fig. 3: Magnified low current region of plot in Fig. 2.
Fig. 4: Plot of voltage vs. current for H2-Air PEM FC electrodes area ratio experiment.
Fig. 5: Magnified low current region of plot in Fig. 4.
The results also showed a consistent change in the open voltage of the cells when the aria ratios were adjusted for stoichiometry compared to when the equal area electrodes were used for cases involving the same oxidizer [see Table 2.] In fact after a period of one, the membrane used had degraded due to other investigations, time and storage condition, yet the drop in open circuit voltage when going from stoichiometric ratio to equal area remained the same. Therefore, in equation (6) and in equation (7) can be found by theoretically solving the change in open circuit voltage. For the H2-O2 PEM fuel cell operation, the equation
Edesired ( Eq.1 used) Eactual ( Eq.6 used) 0.115
(8)
actual ,H O
85.35%
2 2
2 2
and
was solved to get
0.3385
(9)
actual ,H Air 50.26%
2
2
where the potential chemical energy lost to the environment through the H2 and H2O2 has been considered in the calculation.
Similarly, for H2-Air PEM fuel cell operation, the equation
Edesired ( Eq.2 used) Eactual ( Eq.7 used) 0.055 (10) was solved to get
The virtual efficiency may also stand for the limit of efficiency that can be practically observed in alkaline fuel cells, PEM fuel cells and phosphoric acid fuel cells designed with electrodes of equal areas and thickness and operating with air as the oxidizer. The virtual efficiencies obtained were compared with average
0.2971
(11)
maximum efficiencies of practical fuel cells from different sources and it was observed that the maximum efficiencies of practical fuel cells using electrodes of equal aeas and thickness are approximately their virtual efficiencies. Literature have
Table 2: Estimation of open voltage drop due to equal areas
electrodes
reported average maximum efficiency of 40% for PEM, Alkaline and Phosphoric Acid fuel cells [4, 20] and this agrees with virtual 39.69% . The above analysis also indicates that the actual efficiency of practical fuel cell is dependent on the concentration of O2 at the cathode side assuming negligible ohmic losses.
Fuel &
Oxidizer
Stoichiometric Rxn
open circuit voltage Edesired(V)
Equal areas Rxn
open circuit voltage Eactual(V)
E=( Edesired- Eactual)
(V)
Remark
H2-O2
1.02
0.92
0.10
Date: 9/24/2006
H2-O2
0.97
0.84
0.13
Date: 12/21/2007
H2-Air
0.99
0.94
0.05
Date: 9/24/2006
H2-Air
0.84
0.78
0.06
Date: 12/24/2007
Fuel &
Oxidizer
Stoichiometric Rxn
open circuit voltage Edesired(V)
Equal areas Rxn
open circuit voltage Eactual(V)
E=( Edesired- Eactual)
(V)
Remark
H2-O2
1.02
0.92
0.10
Date: 9/24/2006
H2-O2
0.97
0.84
0.13
Date: 12/21/2007
H2-Air
0.99
0.94
0.05
Date: 9/24/2006
H2-Air
0.84
0.78
0.06
Date: 12/24/2007
-
DISCUSSION
Eqs (9) and (11) into Eqs (6) and (7) gives
H2 O2 0.3385H2O2 0.6615H2O 0.33075O2
(12)
H2 0.21O2 0.79N2 0.3533H2O 0.61335H2 0.03335H2O2 0.79N2
(13)
Practically, the only known PEM fuel cell reaction equation is the stoichiometric reaction, so after manufacturing a fuel cell using equal electrodes area, its performance is measured against the desired stoichiometric equation. Therefore, by adopting this method of measure, the energy conversion effectiveness which can be referred to as the virtual efficiency is defined as [26]
The analysis showed that the use of equal electrodes area and thickness has the effect of reducing the efficiency of a fuel cell. This is true because equal electrodes area and thickness, causes excess or insufficient supply of oxidizer at the cathode. For example, hydrogen protons recombine with electrons to form hydrogen gas due to insufficient supply of oxidizer. The hydrogen gas formed at the cathode flows away, burns up and contributes to the waste heat of the fuel cell. The virtual efficiencies obtained for the equal area electrodes in the analysis were compared to values practically obtained from fuel cells, and it was found that the virtual efficiency are more accurate prediction of fuel cell performance. The efficiency of a fuel cell can be increased by incorporating it in a hybrid with a heat engine. In such hybrids, the heat engine runs on the waste heat of the fuel cell and generates additional useful power. But this method of improving works best for fuel cells that operate at high temperature. Adjusting the active electrode area ratio to match the stoichiometric reaction equations, Eqs.
-
and (2), has effect on the performance of the fuel cell as was shown in the experimental result and this effect is positive at low current densities. However, PEM fuel cell does not
virtual
G of actual reaction
H of desired stoichiometric reaction
(14)
benefit much from such a modification at high current densities because of the thin membrane whose nano pores only allows the diffusion of protons in the direction across the membrane.
Therefore, virtual for the actual cell reaction equation (6) is given as
In order to clarify why PEM fuel cell benefits less from electrodes area adjust, consider the schematics in Figs. 7 and 8.
2 2
2 2
virtual,H O
85.35%
At low current densities, the velocity of protons in the x and y direction are approximately equal or of the same scale (ux ~ uy), so the fuel has high performance as desired. At high current
While virtual for the actual cell reaction equation (7) is given as densities, the velocity of protons in the x and y direction are
2
2
virtual ,H Air
34.85%
markedly different. The proton velocity in the x direction is much greater than the velocity in the y direction (ux >> uy), so the effective fuel cell size drops to ACathode for case C
The actual efficiencies are
experiment and AAnode for case D experiment. Consequently, the power capacity drops as observed in the experimental
results. Alkaline fuel cells and phosphoric acid fuel cells may benefit more from this modification, because their electrolytes are in liquid form and ions can easily move in more than one direction.
Fig. 6: Proton velocity and directions for discussion of H2-O2 PEM fuel cell electrodes area ratio experimental result.
Fig. 7: Proton velocity and directions for discussion of H2-Air PEM FC electrodes area ratio experimental result.
Polarization curves by some authors [2, 3] shows that the region where the electrode adjustment positively affect performance indicates that using equal electrode areas in PEM
fuel cells magnifies the activation losses and concentration losses.
Adjusting pressure or flow rates to improve stoichiometry has been observed to offer limited improvement in performance, because of the limited absorption rate of the reactant gases [27, 28], so it was not investigated in this study. Additionally, the PEM fuel cell membrane used for the experiment had been used for over a year so the performance of the fuel cell had reduced due to membrane degradation. However, results are presented to show the differences between the cases investigated as observed in the experiment.
CONLUSSIONS
It was shown theoretically and experimentally that the conventional design of PEM fuel cells with electrodes of equal areas plays a major role in the low efficiency that has been observed in practical fuel cells. It was also shown that stoichiometric condition is desirable in fuel cell controlled combustion for high performance and insufficient or excess oxygen at the PEM fuel cell results in the production of undesirable species on the cathode side. It was also demonstrated that PEM fuel cells operated on H2 have better efficiency than one operated on air due to the effect of stoichiometry.
Adjusting of PEM fuel cells electrodes area ratio to match stoichiometry has limited positive effect because of the thin nature of the PEM.
REFERENCES
-
H. A. Liebhafsky and D. L. Douglas. Introduction; in G. J. Young. Fuel Cell: A Symposium held by the Gas and Fuel Division of the American Chemical Society at the 136th National Meeting in Atlantic City, 1959. New York Reinhold, pp 1-10, 1960.
-
R. OHayre, S. Cha, W. Colella and F. B. Prinz. Fuel Cell Fundamentals, 3rd Ed. John Wiley and Sons, New Jersey, 2016
-
L. J. M. J. Blomen and M. N. Mugerwa. Fuel Cell Systems. Plenum, New York, 1993.
-
G. Hoogers. Fuel Cell Technology Handbook. CRC Press, New York, 2003.
-
M. Paster, Presidents Hydrogen Fuel Initiative. US Department of Energy Publication, August 2005;
<https://www.energy.gov/sites/pro/file/2014/03/f12/hpwgw_doe_paster. pdf>; Accessed 03/08/2020
-
ydrogen Economy Fact Sheet: US-EU Summit on the Cooperation on the Development of a Hydrogen Economy. President George W Bush administration Press Release, June 25, 2003;
<https://georgewbush- whitehouse.archives.gov/news/release/2003/06/20030625-6.html>;
Accessed 03/08/2020
-
W. T. Grubb and L. W. Niedrach. Batteries with solid ion-exchange membrane electrolytes, II: low temperature hydrogen-oxygen fuel cells
Journal of the Electrochemical Society, 107, pp 131135, 1960
-
G. A. Eisman. The Application of Dow Chemicals Perfluorinated Membranes in Proton-Exchange Membrane Fuel Cells, Journal of Power Sources, 29, pp 389-398, 1990.
-
B. R. Ezzel, B. Carl and W. A. Mod. Ion Exchange Membranes for the Chlor-Alkali Industry, AIChE Symposium Series, Houston, pp 49-51, March 1985.
-
G. A. Eisman. The Application of a New Perfluorosulfonic Acid Ionomer in Proton-Exchange Processes, 136th Proceeding of the Electrochemical Society Meeting, Boston. May, 1986
-
M. Rikukawa, and K. Sanui. Proton-Conducting Polymer Electrolyte Membranes based on Hydrocarbon Polymers, In Brandon NP, Fuel Cells Compendium. Elsevier 2005
-
D. E. Curtis, R. D. Lousenberg, T. J. Henry, P. C. Tangerman and M. E. Tisack. Advanced Materials for Improved PEMFC Performance and Life, In Brandon NP, Fuel Cells Compendium. Elsevier 2005
-
S. Litster and G. McLean. PEM Fuel Cell Electrodes, In Brandon NP,
Fuel Cells Compendium. Elsevier 2005
-
V. Mehta and J. S. Cooper. Review and Analysis of PEM Fuel Cell Design and Manufacturing, In Brandon NP, Fuel Cells Compendium.
Elsevier 2005
-
M. Khakpour and K. Vafai. Analysis of Transport Phenomena within PEM Fuel Cells An Analytical Solution, International Journal of Heat and Mass Transfer 2008, 51, pp 3712-3723.
-
X. Li and I. Sabir. Review of Bipolar Plates in PEM Fuel Cells: Flow- field Designs, International Journal of Hydrogen Energy, 30, pp 359- 371, 2005
-
R. R. Passos, V. A. Paganin and E. A. Ticianelli. Studies of the Performance of PEM Fuel Cell Cathodes with the Catalyst Layer Directly applied on Nafion Membranes, Electrochemica Acta, 51, pp 5239-5245, 2006.
-
C. Song, Y. Tang, J. L. Zhang, J. Zhang, H. Wang, J. Shen, S. McDermid, J. Li and P. Kozak. PEM Fuel Cell Reaction Kinetics in the Temperature Range 23-120 , Electrochemica Acta, 52, pp 2552- 2561, 2007.
-
J. Zhang, C. Song, J. Zhang, R. Baker and L. Zhang. Understanding the effects of Backpressure on PEM Fuel Cell Reactions and Performance, Journal of Electroanalytical Chemistry, 688, pp 130-136, 2013.
-
M. M. Hussain, J. J. Baschuk, X. Li and I. Dincer. Thermodynamic Analysis of a PEM Fuel Cell Power System, International Journal of Thermal Sciences, 44, pp 903 911, 2005.
-
X. Zhang, J. Guo, and J. Chen. The Parametric Optimum Analysis of a Proton Exchange Membrane (PEM) Fuel Cell and its Load Matching, Energy, 35, pp 5294 5299, 2010.
-
F. Barbir and T. Gomez. Efficiency and Economics of Proton Exchange Membrane (PEM) Fuel Cells, International Journal of Hydrogen Energy, 22 (10/11), pp 1027 1037, 1997.
-
W. H. Zhu, R. U. Payne and B. J. Tatarchuk. Critical Flow Rate of Anode Fuel Exhaust in a PEM Fuel Cell System, Journal of Power Sources, 156, pp 512 519, 2006.
-
M. H. Akbari and B. Rismanchi. Numerical Investigation of Flow Field Configuration and Contact Resistance for a PEM Fuel Cell Performance, Renewable Energy, 33, pp 1775 1783, 2008.
-
V. A. Sethurama, J. W. Weidner, A. T. Haug, M. Pemberton and L. V. Protsailo. Importance of Catalyst Stability vis-avis Hydrogen Peroxide Formation Rates in PEM Fuel Cell Electrodes, Electrochemica Acta, 54, pp 5571 5582, 2009.
-
Udoetok, Etim Sunday, "An investigation into fuel cells and flow cytometers optimal design, LSU Doctoral Dissertations. 1638, 2008.
<https://digitalcommons.lsu.edu/gradschool_dissertations/1638>
-
W. H. Zhu, R. U. Payne and B. J. Tatarchuk. Critical Flow Rate of Anode Fuel Exhaust in a PEM Fuel Cell System, Journal of Power Sources, 156, pp 512 519, 2006.
-
M. H. Akbari and B. Rismanchi. Numerical Investigation of Flow Field Configuration and Contact Resistance for a PEM Fuel Cell Performance, Renewable Energy, 33, pp 1775 1783, 2008.