
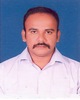
- Open Access
- [post-views]
- Authors : Dr. N Dhachanamoorthi, Dr. M. Jothi, S. Tamilselvan
- Paper ID : IJERTV8IS120339
- Volume & Issue : Volume 08, Issue 12 (December 2019)
- Published (First Online): 14-03-2020
- ISSN (Online) : 2278-0181
- Publisher Name : IJERT
- License:
This work is licensed under a Creative Commons Attribution 4.0 International License
Synthesis and Characterization of Polypyrrole-Antimony (III) Oxide Hybrid Polymer Nanocomposites
Dr. N Dhachanamoorthi1
1Assistant Professor & Head,
Dr. M. Jothi2
2Assistant Professor,
PG Department of Physics, Vellalar College for Women, PG Department of Physics, Vellalar College for Women, Erode, Tamilnadu, India. Erode, Tamilnadu, India.
S. Tamilselvan3 3Assistant Professor of Physics, Nandha Arts and Science College,
Erode, Tamilnadu, India
Abstract-The PPy-Sb2O3 nanocomposites with Sb2O3 (< 250 nm) nanoparticles various weight percents were prepared by mechanical mixing. Fourier transform infrared (FTIR) spectroscopy, ultraviolet-visible (UVVis) spectroscopy, X-ray diffraction (XRD), thermogravimetric analysis (TGA), differential scanning calorimetry (DSC), scanning electron microscopy (SEM), and energy dispersive X-ray analysis spectroscopy (EDAX) were used to characterize the PPy-Sb2O3 nanocomposites. The FTIR results indicated that there are some interactions between PPy and Sb2O3 nanoparticles. Such an interaction is likely caused by the formation of the coordinate bonding between the lone pair electron of atom in PPy chain with orbit of Sb atom of Sb2O3, indicating a reduction in the strength of PPy-Sb2O3 interactions as the wt % increases, which may lead to the broader size distribution of Sb2O3 nanoparticles dispersed in nanocomposites.
Schematic diagram PPy-Sb2O3 nanocomposites
The UV-vis results of PPy-Sb2O3 nanocomposites interactions is significantly increased by increasing the Sb2O3 wt%, leading to reduce the energy level interval of benzenoid ring and hence result in a red shift. The XRD result indicates that PPy has been successfully anchored on the surface of Sb2O3 nPs through the mechanical mixing method. The morphology and elemental composition analysis were characterized by using SEM and EDAX. This result indicates high interaction between PPy and metal oxides.
-
INTRODUCTION
Hybrid inorganic-organic nanocomposite materials have attracted more and more attention due to creating new
materials which combine different functions and characteristics of individual materials. Different inorganic materials including carbon nanotubes, metal, metal oxides and nanosheets have been investigated in polymer matrices [1]. Nanocomposite materials have attracted a lot of interest due to their probable commercial exploitation as sensors, batteries, toners in copying machines, quantum electronic devices, smart windows and materials for electromagnetic shielding, etc. Nanocomposite materials made from nanoparticles of oxides and conducting polymers are the most interesting and challenging areas of research in recent times [2]. The conducting polymers, such as polythiophene, polypyrrole (PPy) and polyaniline have been exhaustively studied due to their outstanding mechanical and electrical properties, which afford applications in actuators, sensors and electrochromic devices [3]. Among the conducting polymers, PPy has attracted considerable attention because it is easy synthesis, it has relatively good quality environmental stability and its surface charge characteristics are can be customized by changing the dopant species into the material during the synthesis [4,5]. In the present work, we report the fabrication of conductive PPy-Sb2O3 nanocomposites using mechanical mixing method. The chemical structures of the PPy-Sb2O3 nanocomposites are characterized by Fourier transform infrared (FT-IR) spectroscopy and optical parameters are by using UV-vis characterization. The thermal stability of the PPy-Sb2O3 nanocomposites is performed by thermogravime – tric analysis (TGA) and differential scanning calorimetric (DSC). Scanning electron microscope (SEM) is used to characterize the dispersion of Sb2O3 nPs and the morphology of the PPy-Sb2O3 nanocomposites. The effects of the Sb2O3 nPs on the crystallization structures of the PPy are also studied. In this present work, the inorganic-organic hybrid nanocomposite containing polypyrrole as the organic part and antimony (III) oxide as the inorganic part have been used for studying structural, optical and thermal properties. Such types of nanocomposite have shown to posses small grain size and high stability. To the best of our knowledge, this is the first
ever attempt made to synthesis and investe of these nanocomposites with special properties.
-
EXPERIMENTAL
-
Materials
All of the chemical reagents used in this experiment were A.R. grade. The monomer pyrrole (PPy) and Dodecylbenzene Sulfonic acid (DBSNa) as dopant was purchased from Aldrich Chemical and purified by distillation under reduced pressure, stored in refrigerator before use. Antimony (III) oxide nanopowder, <250 nm nanoparticle (98% purity) from Aldrich Chemical, Ammonium peroxydisulfate (99%, Merck), Ethonal (99% purity, Merck), Acetone (99% purity, Merck) were purchased from Merck chemical. The water used throughout the work is distilled water.
-
The preparation of polypyrrole (PPy)
The polypyrrole was synthesized by chemical oxidation polymerization under static condition in a lower temperature. About 900 ml of de ionized water was taken in a flask and an arrangement for mechanical stirring. Dodecylbenzene sulfonic acid solution (DBSNa) as a dopant was dissolved in above 900 ml of deionized water and the solution was well stirred in the flask. Monomer pyrrole was added in the above suspension solution and keeps stirring for 30min. After 30 min ammonium peroxydisulfate (NH4)2S2O8 as an oxidant was added drop wise slowly to the good degree of polymerization is achieved the suspension solution was dark black in color. The entire solution mixture was continuously stirred well at 0-5C and the reaction was continued for another 24 h over all time speed of rotation maintained at 700rpm. The product was filtered and washed with deionized water, ethanol and acetone, then dried under vacuum at 80C for 24 h. Experimental setup as shown in Fig.1.
-
Synthesis of polypyrrole-Sb2O3 nanocomposites
PPy-Sb2O3 nanocomposites were synthesized using different wt% of Sb2O3 with respect to polypyrrole which are referred as PPy-Sb2O3 nanocomposites. Pure PPy was synthesized following the same procedure without Sb2O3 nanoparticles. The molar ratio of polymer (PPy) and metal Oxide Sb2O3 was 1:0.25 to prepare PPy-Sb2O3 (25%) nanocomposites by using mechanical mixing method. Similarly the samples were prepared in the different weight % of Sb2O3 nanoparticles like PPy-Sb2O3 (50%) and PPy-Sb2O3 (100%) by the ratio 1:0.50
and 1:1 respectively.
-
-
CHARACTERIZATION
FT-IR spectra of the pure PPy, PPy-Sb2O3 nanocomposites, Sb2O3 nPs samples were recorded at room temperature using FT-IR Spectrometer Make: Perkin Elmer; Model: Spectrum RX 1; Range: 400 cm-1-4000 cm-1; Resolution: 4. The sample was prepared in the pellet form by mixing the polymer powder with KBr by the ratio 1:10 and pressing it in the Perkin Elmer hydraulic device using 15 ton pressure.
Fig.1 Experimental setup for chemical oxidative polymerization method
UV-vis spectra of the synthesized PPy-Sb2O3 nanocomposites powder were determined using a UVvis spectrometer, Model: Lambda 35; Range: 400 nm-1100 nm; Resolution: 4; Mode of operation: 1.Transmittance (T %) and Absorbance (A %) 2. Reflectance (R %). X-ray diffraction patterns of PPy-Sb2O3 nanocomposites samples performed using advance diffractometer with monochromatic CuK radiation (=1.54Ã…) are used to identify crystalline nature of the samples. The crystallite size was determined from Scherre relation.
(1)
where, D is the crystallite size, K is the shape factor for the average crystallite (w0.9), is the wavelength of the X-ray which is 1.54 Å for Cu target, B is the full width at half maxima of the crystalline peak in radians, is the angle between incident and reflected rays. Thermogravimetric properties of the pure PPy and PPy-Sb2O3 nanocomposites were studied in instrument used: STA449 F3 Jupiter; Temperature range: RT to 500 ºC; Heating rate: 10K/min; Atmosphere: Nitrogen; Sample Carrier: TG-DSC Sample Carrier; Sample Crucible: TG-DSC Alumina Crucible with lid. The microstructure, size and morphology of the synthesized polypyrrole as well as their dispersity in the PPy- Sb2O3 nanocomposites could be determined with the help of scanning electron microscopy (SEM) images were obtained on Make: JEOL; Modal: JSM 6390; Made in Japan. Energy dispersive X- ray spectroscopy employed to analyze the chemical compositions of nanocomposites was carried out using Make: Oxford Instruments; Modal: INCA Pental FET 3; Made in England.
-
RESULT AND DISCUSSION
4.1 FTIR spectral analysis
The FTIR spectra of pure PPy, PPy-Sb2O3 (25-100%) nanocomposites and pure Sb2O3 nPs are shown in Fig.2. The main transmittance peaks of PPy are appeared at 3402.05 cm-1 and 1547.23 cm-1 could be corresponded to the N-H stretching vibration and symmetric stretching vibration of C-C bond in the PPy ring, respectively. The band at 1397.53 cm-1 is assigned to N-H bending vibration bond. The transmittance
peaks appeared at 1184.22 cm-1 and 905.96 cm-1 was attributed to the in-plane bending vibration of C-H bond and the CH out-of-plane bending vibration indicating the polymerization of pyrrole respectively [6]. The FTIR spectrum of the PPy-Sb2O3 (25%) nanocomposite demonstrated the peaks at 3396.57 cm-1, 1545.98 cm-1, 1381.84 cm-1, 1185.86
cm-1, and 908.86 cm-1 that are considered to arise from pyrrole ring stretching, N-H stretching vibration, C-C symmetric stretching vibration, N-H bending vibration, C-H in plane bending vibration and C-H out-of-plane bending respectively. The transmittance peaks and corresponding stretching vibration of pure PPy, PPy-Sb2O3 nanocomposites was shown in Table.1. These results confirmed the presence of PPy moieties in the nanocomposites. Interestingly, all peak positions shifted towards higher values after Sb ions adsorption. The delocalized electrons in PPy matrix, which are involved in the skeletal vibration of PPy ring, are affected by the doping ions in the polymer matrix. Different types of dopants in the PPy backbone may disturb the conjugate structure of PPy and this limit the extent of charge delocalization along the polymer chains, leading to red shift. However, as for PPy-Sb2O3 nanocomposites, except the peaks of PPy, the broad band between 500 and 950cm-1 are
Fig.2 FTIR spectra of pure PPy (a) and PPy-Sb2O3 nanocomposites (b, c & d)
Sample Name
N-H
stretching vibrations (cm-1)
CC ring symmetric stretching vibrations (cm-1)
N-H
bending vibrations (cm-1)
In-plane CH
bending Vibrations (cm-1)
Out-plane CH
bending Vibrations (cm-1)
Pure PPy
3402.05
1547.23
1397.53
1184.22
905.96
PPy- Sb2O3 (25%)
3396.57
1545.98
1381.84
1185.86
908.86
PPy- Sb2O3 (50%)
3410.94
1548.74
1387.97
1187.47
911.61
PPy- Sb2O3 (100%)
3373.71
1544.73
1395.43
1181.12
916.23
Sample Name
N-H
stretching vibrations (cm-1)
CC ring symmetric stretching vibrations (cm-1)
N-H
bending vibrations (cm-1)
In-plane CH
bending Vibrations (cm-1)
Out-plane CH
bending Vibrations (cm-1)
Pure PPy
3402.05
1547.23
1397.53
1184.22
905.96
PPy- Sb2O3 (25%)
3396.57
1545.98
1381.84
1185.86
908.86
PPy- Sb2O3 (50%)
3410.94
1548.74
1387.97
1187.47
911.61
PPy- Sb2O3 (100%)
3373.71
1544.73
1395.43
1181.12
916.23
attributed to the Sb-O bond, suggesting that the Sb2O3 was
embedded in PPy matrix. The results indicated that there are some interactions between PPy and Sb2O3 particles. Such an interaction is likely caused by the formation of the coordinate bonding between the lone pair electron of atom in PPy chain with orbit of Sb atom of Sb2O3, indicating the strength of PPy- Sb2O3 interactions, as the wt % increases, which may lead to the broader size distribution of Sb2O3 particles dispersed in nanocomposites. Compared to pure PPy, the characteristic
peaks of PPy-Sb2O3 nanocomposites slightly shifted to higher
wavelength, indicating the strong interaction at the interface. Besides, the characteristic peaks of PPy-Sb2O3 are well maintained in the nanocomposites, indicating that PPy has been successfully compounded with Sb2O3 without changing chemical composition. Comparing to the corresponding peaks of pure PPy, the peaks of PPy-Sb2O3 shifted towards lower wavenumber. This shifting of absorption bands may be due to the action of hydrogen bonding between the hydroxyl groups on the surface of Sb2O3 nPs and the amine groups in the PPy molecular chains. Similar observations of absorption shifting peaks of PPy-Sb2O3 towards are obtained in lower wavenumber. This result indicates that the PPy-Sb2O3 nanocomposites have been successful synthesized and the observed shift indicates the interaction between PPy and nPs.
Table.1 FTIR data of pure PPy and PPy-Sb2O3 nanocomposites
-
UV-vis absorption spectral analysis
The UV-vis spectra of pure PPy (a), PPy-Sb2O3 (b,c, d) nanocomposites and pure Sb2O3 (e) are shown in Fig.3. The absorption reveals that there is different composition and morphology in ranging Sb2O3 concentration from 25-100% in PPy-Sb2O3 nanocomposites. However, as the characteristic absorption bands of pure PPy are obtained in the wavelengths range of 250-300 nm, 450-450 nm and 900-1000 nm. UV-vis analysis was also conducted to analyze the PPy are presented in Fig.4 (a), in which the intermediates exhibit an absorption band appeared at about 473nm. This band is due to the formation of phenazine-like structures in this stage. These bands are assigned to the formation of PPy. The first absorption band corresponds to the -* electron transition within benzenoid segments. The second and the third bands correspond to the doped state and the polaron formation in PPy respectively. From the spectroscopic and theoretical data indicate, that the absorption band at 400-500 nm (4-3 eV) is assigned to -* transition of PPy. The band gap of each case is determined from Tauc plot which is shown in Table.2.
(1)
Fig.3 UV-vis spectra of pure PPy (a), PPy-Sb2O3 nanocomposites (b, c, d) and pure
Sb2O3 nPs
Sample Name
Wavelength (nm)
Absorption
Band gap (eV)
Band: 1
Band: 2
Band: 3
Band: 1
Band: 2
Band: 3
Pure PPy
260
473
931
0.1826
0.1581
0.2017
3.46
PPy- Sb2O3 (25%)
343
360
379
0.3393
0.4155
0.3951
3.04
PPy- Sb2O3 (50%)
342
359
379
1.0751
1.4434
1.3211
1.76
PPy- Sb2O3 (100%)
343
359
380
1.7405
1.7130
1.8569
1.49
Pure Sb2O3
340
359
380
1.8181
2.0666
2.5913
1.71
Table.3 Crystallographic parameters of pure PPy, PPy-Sb2O3 nanocomposites and pure Sb2O3 nPs
Among the various cases, the highest band gap energy was obtained for pure PPy and then the band gap was decreased with increasing Sb2O3 concentration which is clearly shown in the Table.2. The PPy-Sb2O3 interactions is significantly increased by increasing the Sb2O3 wt%, leading to reduce the energy level interval of benzenoid ring and hence, result in a red shift. The FTIR spectra of the nanocomposites shown in Fig.3 also support this conclusion. Upon doping PPy exhibits unusual electronic structure due to electronphonon coupling. Polarons and bipolarons states appear within the band gap, which gives rise to the broad band at wave length 900-1000 nm in the case of pure PPy. Generally, the optical band gap in a semiconductor is determined by plotting absorption coefficients () as (h)1/m vs. h where m represents the nature of the transition and h is the photon energy. Now m may have different values, such as , 2, and 3 for allowed direct, allowed indirect, forbidden direct and forbidden indirect transitions respectively.
where A is the absorption constant for a direct transition. For allowed direct transition one can plot (h)2 vs. h as shown in Fig.4 and extrapolate the linear portion of it to =0 value to obtain the corresponding band gap.
Fig.4a
The optical absorption coefficient () near the absorption edge for direct interband transitions is given by the equation (1). The band gap of PPy with antimony concentration implies that electronic structure of PPy is affected [8,9]. UV-Vis spectral data and the band gap of pure PPy, PPy-Sb2O3 (25-100%) nanocomposites and pure Sb2O3 are as shown in Table.2.
Fig.4b
Fig.4c
Fig.4d
Fig.4d
Fig.4e
Fig.4a-4e Tauc plot for (h)2 vs h of pure PPy, PPy-Sb2O3 nanocomposites and pure Sb2O3 nPs
-
X-Ray diffraction studies
Fig.5 shows the XRD patterns of pure PPy, PPy- Sb2O3 nanocomposites (25-100%) and pure Sb2O3 nPs which is a evidence of crystalline nature of the samples. The XRD pattern of pure PPy shows a broad peak and sharp peak appeared at 23.04º and 44.36º respectively, which indicates the crystalline nature. XRD curve of PPy shows that the PPy prepared in the absence of Sb2O3 nPs is amorphous in nature. The crystallite sizes of the PPy were estimated from X-ray line broadening using Scherer's formula. It can be seen clearly from the XRD patterns of Sb2O3 nPs, that the Sb2O3 nPs showed a single-phase in nature. There was no secondary phase detected and the high intensity of the peaks revealed the crystalline nature of the as Sb2O3 nPs. Obviously, the diffraction peaks of the Sb2O3 nPs appear in the PPy-Sb2O3 nanocomposites from the Fig.5 (b,c,d) the intensity of these peaks becomes stronger with increasing the nanoparticle loadings, while the two original peaks of PPy show a reduced intensity at 2=23.04 and 44.36º. The XRD pattern also confirm the presence of antimony in the PPy-Sb2O3 (25-100%) nanocomposites and pure Sb2O3 the crystallize size as-calculated, where the average crystallize size are 97 nm (25%), 170 nm (50%), 176 nm (100%) and 152 nm
(pure Sb2O3). The strain and dislocation density of pure PPy, PPy-Sb2O3 nanocomposites (25-100%), pure Sb2O3 nPs data are seen in Table.3. The parameters are slightly changed with the addition of Sb2O3 nPs. Furthermore, these results revealed the amorphous nature of PPy in the nanocomposites, suggesting that the addition of Sb2O3 nPs retain the crystallization of the PPy molecular chains. This may be because when PPy is adsorbed on the surface of the Sb2O3 nPs. The increasing trend intensity indicating that the Sb2O3 greatly increased due to the adsorption of PPy molecular chains on the surface of the Sb2O3 nPs. In order to study the effect of the addition of Sb2O3 nPs in PPy matrix, a careful analysis of the position of the XRD peak indicates that, there is a shifting in peak's position towards lowering 2 value, but in this case, the crystallinity of Sb2O3 nPs was found to be disturbed in the PPy-Sb2O3 nanocomposites. However, in the present work the crystallinity of Sb2O3 is not disturbed by PPy
molecular chain on the surface of Sb2O3 nPs as can be seen from Fig.6. The shifting of the peak's position clearly indicates that PPy-Sb2O3 nanoparticles are incorporating into the PPy polymer matrix. The broad weak diffraction peak of PPy still exists, but its intensity decreases. This indicates a strong effect of the Sb2O3 nPs on the structures of crystalline of the formed PPy and the interaction between PPy backbone and Sb2O3 nPs.
Fig.5 X-Ray diffraction patterns of pure PPy (a), PPy-Sb2O3 nanocomposites (b, c, d) and pure Sb2O3 nPs (e)
This result indicates that, PPy has been successfully anchored on the surface of Sb2O3 NPs through the mechanical mixing method. However, the characteristic peak intensities of PPy-Sb2O3 nanocomposite gradually decreased with increasing the weight percentage of Sb2O3, indicating the incorporation of Sb2O3 into the polymer matrix. Previous literature also support that the parent work that the introduction of Sb2O3 will affect the crystalline behavior of PPy [10-12].
Pure PPy
2
FWHM
Intensity
d Spacing Value
Crystallize size (nm)
Strain
Dislocation density
1
23.04
0.071
263
3.8570
114
0.0006
1.30*10-14
2
44.36
0.094
980
2.0404
91
0.0004
8.32*10-15
Peak No
PPy-Sb2O3 (25%)
2
FWHM
Intensity
d Spacing Value
Crystallize size (nm)
Strain
Dislocation density
1
13.88
0.118
130
6.3749
67
0.0018
0.20*10-15
2
27.80
0.118
1647
3.2065
69
0.0009
4.80*10-15
3
28.52
0.118
220
3.1271
69
0.0009
4.80*10-15
4
32.22
0.165
610
2.7760
50
0.0001
2.50*10-15
5
35.20
0.071
140
2.5475
117
0.0004
1.37*10-14
6
46.10
0.118
570
1.9673
73
0.0005
5.34*10-15
7
54.64
0.071
17
1.6783
125
0.0002
1.58*10-14
8
57.26
0.118
170
1.6076
76
0.0004
5.87*10-15
9
59.14
0.071
103
1.5609
128
0.0002
1.65*10-14
10
64.14
0.118
57
1.4507
79
0.0003
6.30*10-15
11
68.96
0.071
77
1.3606
135
0.0002
1.84*10-14
12
74.08
0.071
163
1.2787
140
0.0001
1.96*10-14
13
76.44
0.071
170
1.2450
142
0.0001
2.02*10-14
Peak No
PPy-Sb2O3 (50%)
2
FWHM
Intensity
d Spacing Value
Crystallize size (nm)
Strain
Dislocation density
1
14.00
0.047
213
6.3205
170
0.0007
2.89*10-14
2
27.94
0.047
1747
3.1907
174
0.0003
3.03*10-14
3
28.76
0.047
113
3.1016
174
0.0003
3.04*10-14
4
32.34
0.071
567
2.7659
116
0.0004
1.35*10-14
5
35.28
0.071
153
2.5419
117
0.0004
1.37*10-14
6
46.24
0.071
607
1.9617
121
0.0003
1.47*10-14
7
54.80
0.047
533
1.6738
190
0.0001
3.62*10-14
8
57.42
0.047
173
1.6035
192
0.0001
3.71*10-14
9
59.32
0.071
110
1.5566
128
0.0002
1.65*10-14
10
64.28
0.047
83
1.4479
199
0.0001
3.98*10-14
11
69.04
0.047
80
1.3593
205
0.0001
4.20*10-14
12
74.20
0.047
153
1.2770
211
0.0001
4.48*10-14
13
76.60
0.047
103
1.2428
215
0.0001
4.63*10-14
Peak No
PPy-Sb2O3 (100%)
2
FWHM
Intensity
d Spacing Value
Crystallize size (nm)
Strain
Dislocation density
1
13.88
0.047
153
6.3749
170
0.0007
2.89*10-14
2
27.80
0.047
1907
3.2065
174
0.0003
3.02*10-14
3
28.48
0.047
210
3.1314
174
0.0003
3.03*10-14
4
32.20
0.047
713
2.7776
175
0.0003
3.09*10-14
5
35.26
0.047
107
2.5433
177
0.0002
3.14*10-14
6
46.10
0.047
733
1.9673
183
0.0002
3.37*10-14
7
54.30
0.047
47
1.6880
189
0.0001
3.60*10-14
8
57.28
0.094
220
1.6071
96
0.0003
9.26*10-15
9
59.20
0.047
140
1.5595
194
0.0001
3.77*10-14
10
64.38
0.047
53
1.4459
199
0.0001
3.98*10-14
11
68.90
0.047
87
1.3617
203
0.0001
4.19*10-14
12
74.10
0.071
183
1.2784
140
0.0001
1.96*10-14
13
76.32
0.047
123
1.2467
214
0.0001
4.61*10-14
Peak No
Pure Sb2O3
2
FWHM
Intensity
d Spacing Value
Crystallize size (nm)
Strain
Dislocation density
1
13.80
0.047
253
6.4117
170
0.0007
2.89*10-14
2
27.72
0.118
2957
3.2155
69
0.0009
4.80*10-15
3
28.42
0.071
430
3.1379
115
0.0005
1.33*10-14
4
32.10
0.094
1037
2.7861
87
0.0006
7.72*10-15
5
35.06
0.047
273
2.5573
177
0.0002
3.13*10-14
6
46.02
0.094
1130
1.9760
91
0.0004
8.42*10-15
7
54.56
0.047
980
1.6806
190
0.0001
3.61*10-14
8
58.84
0.047
67
1.5681
193
0.0001
3.76*10-14
9
59.14
0.071
80
1.5609
128
0.0002
1.65*10-14
10
64.10
0.071
90
1.4516
131
0.0002
1.74*10-14
11
67.22
0.047
70
1.3916
202
0.0001
4.11*10-14
12
74.04
0.047
263
1.2793
211
0.0001
4.47*10-14
13
76.24
0.047
103
1.2478
214
0.0001
4.61*10-14
Table.3 Crystallographic parameters of pure PPy, PPy-Sb2O3 nanocomposites and pure Sb2O3 nPs
-
Thermogravimetric analysis
The thermogravimetric analysis of pure PPy, PPy- Sb2O3 (25-100%) nanocomposites and pure Sb2O3 nPs is shown in Fig.6. To investigate the weight loss of the as- synthesized pure PPy, PPy-Sb2O3 (25-100%) nanocomposites and pure Sb2O3 nPs samples, the thermogravimetric analysis has been carried out in a nitrogen atmosphere. In order to see the effect of temperature on the thermal behavior of the polymer thermogravimetric analysis of PPy-Sb2O3 nanocomposites has been carried out from 25-500 ºC temperature. To investigate the thermal properties and the interaction between PPy and Sb2O3, TGA analysis has been carried out through decomposition curve. From the Fig.6 (a) pure PPy undergoes two-step decompositions are observed. The first one is appeared at 110 ºC which is due to the removal of adsorbed water resulting with a weight loss of 53.99%. The second step of decomposition starts from 200 ºC and goes up to 450 ºC with about 26.31% weight loss. Finally the residual mass and residual temperature of pure PPy is 19.70 and
497.8 ºC. Degradation of PPy-Sb2O3 (25%) nanocomposite takes place in five steps. The first three steps of weight loss observed at 200 ºC is due to the removal of adsorbed water and the remaining second step (between 200 ºC and 450 ºC) of weight loss is due to the breakdown of the polymer backbone in the nanocomposites as shown in Fig. 7 (b).
Pure PPy
Mass Change
Mass
Residual Mass
Residual Temp
Stage: 1
-53.99
Stage: 2
-26.31
Stage: 3
–
19.70
497.80
Stage: 4
–
Stage: 5
–
PPy-Sb2O3 (25%)
Mass Change
Mass
Residual Mass
Residual Temp
Pure PPy
Mass Change
Mass
Residual Mass
Residual Temp
Stage: 1
-53.99
Stage: 2
-26.31
Stage: 3
–
19.70
497.80
Stage: 4
–
Stage: 5
–
PPy-Sb2O3 (25%)
Mass Change
Mass
Residual Mass
Residual Temp
Fig.6 TGA spectra of pure PPy (a), PPy-Sb2O3 nanocomposites (b,c,d) and pure Sb2O3 nPs (e)
Stage: 1
-3.07
Stage: 2
-2.02
Stage: 3
-4.03
70.63
497.70
Stage: 4
-4.31
Stage: 5
-15.94
PPy-Sb2O3 (50%)
Mass Change
Mass
Residual Mass
Residual Temp
Stage: 1
-3.21
Stage: 2
-1.97
Stage: 3
-4.32
73.15
498.00
Stage: 4
-3.73
Stage: 5
-13.62
PPy-Sb2O3 (100%)
Mass Change
Mass
Residual Mass
Residual Temp
Stage: 1
-1.23
Stage: 2
-0.43
Stage: 3
-1.62
85.06
497.08
Stage: 4
-1.59
Stage: 5
-2.08
Stage: 6
-7.99
Pure Sb2O3
Mass Change
Mass
Residual Mass
Residual Temp
Stage: 1
-3.41
Stage: 2
-3.51
Stage: 3
-4.20
88.88
497.90
Stage: 4
–
Stage: 5
–
Stage: 6
–
Stage: 1
-3.07
Stage: 2
-2.02
Stage: 3
-4.03
70.63
497.70
Stage: 4
-4.31
Stage: 5
-15.94
PPy-Sb2O3 (50%)
Mass Change
Mass
Residual Mass
Residual Temp
Stage: 1
-3.21
Stage: 2
-1.97
Stage: 3
-4.32
73.15
498.00
Stage: 4
-3.73
Stage: 5
-13.62
PPy-Sb2O3 (100%)
Mass Change
Mass
Residual Mass
Residual Temp
Stage: 1
-1.23
Stage: 2
-0.43
Stage: 3
-1.62
85.06
497.08
Stage: 4
-1.59
Stage: 5
-2.08
Stage: 6
-7.99
Pure Sb2O3
Mass Change
Mass
Residual Mass
Residual Temp
Stage: 1
-3.41
Stage: 2
-3.51
Stage: 3
-4.20
88.88
497.90
Stage: 4
–
Stage: 5
–
Stage: 6
–
-
Differential scanning calorimetric analysis
Differential scanning calorimetric spectrum of pure PPy, PPy-Sb2O3 (25-100%) nanocomposites and pure Sb2O3 nPs were shown in Fig.8. Fig. 7 (a) shows the DSC of pure PPy have broad endothermic peak appeared at around 370.3
ºC, this peak reveals the removel of water molecules from the pure PPy molecules. DSC spectrum of pure PPy have sharp exothermic peaks appeared, exothermic peak was shown at about 95.4 ºC, which was the complex peak, area of this peak is 756.5 J/g; the onset and endset temperature of the complex peak is 65.2 ºC, 110.3 ºC respectively, this is presumably due to the polymer decomposition [15].
Fig.7a
Table.4 TGA parameters of pure PPy, PPy-Sb2O3 nanocomposites and pure Sb2O3 nPs
The residual mass and residual temperature of PPy-Sb2O3 (25%) nanocomposite is 70.63 and 497.7 ºC respectively. Mass changes, residual mass, residual temp of pure PPy, PPy- Sb2O3 (25-100%) nanocomposites and pure Sb2O3 nPs are shown in Table.4.The mass change of PPy-Sb2O3 (25-100%) nanocomposites have five stages of weight loss are obtained for this nanocomposites while the spectrum pure Sb2O3 nPs have two weight loss appeared in which the weight loss is decreased with increasing Sb ion concentration and the residual mass of PPy-Sb2O3 (25-100%) nanocomposites are 70.63 (25%), 73.15 (50%), 85.06 (100%). This data clearly
reveals the residual mass are increased Sb ion content and with have constant residual temperature [13,14]. By comparing the thermo graphs of synthesized are pure PPy and nanocomposites, one can be understood, the different thermal behavior of the materials. The residual mass increased with the ionic concentration of Sb2O3 in PPy-Sb2O3 nanocomposite materials. These results show that the PPy-Sb2O3 nanocomposites materials have remarkable improvement in thermal stability. These results confirm the strong interaction between polypyrrole and Sb2O3 forming a stable nanocomposites.
Fig.7b
Fig.7c
Fig.7d
Fig.7e
Fig.7a-7e DSC spectra of pure PPy, PPy-Sb2O3 nanocomposites and pure Sb2O3 nPs
The DSC spectrum of PPy-Sb2O3 (25-100%) nanocomposites is shown in Fig.7 (b-d). The exothermic peaks
appeared in the PPy-Sb2O3 spectrum which named the complex peaks. From PPy-Sb2O3 (25-100%) nanocomposites spectrum which have complex peaks and the area of this complex peaks are 5.481 J/g, 17.150 J/g, and 94.200 J/g. To compare these nanocomposites, the area of the complex peaks is increased with increasing the Sb2O3 concentration. The peaks indicating that, the polymer decomposition was found to be present in all ratios (25-100%) of nanocomposites. The peaks indicating that the polymer decomposition was found to be present in PPy-Sb2O3 (25-100%), but that was clearly absent in pure Sb2O3 nPs samples. Despite the degradation of PPy-Sb2O3 (25-100%) nanocomposites samples indicating the gradual enhancement of thermal stability of the polymer chain with increasing the amount of Sb2O3. The exothermic peak disappeared for pure Sb2O3 sample, indicating strong interaction of the oxide with the polymer chain [16].
-
Scanning electron microscopic studies
Scanning electron microscopy (SEM) images of the pure PPy, PPy-Sb2O3 (25-100%) nanocomposites and pure Sb2O3 nPs are shown in Fig. 8(a-e). The micrographs of pure PPy powder (Fig.8a) show big globular clusters of polymers. The surface morphology of pure PPy changed completely, when it was converted to the nanocomposites with Sb2O3 (Fig.8b-d), which established the interaction of Sb2O3 surface with the polymer chain. The white colour is Sb2O3 nPs and light coloured shell is PPy in the nanocomposites. The prepared nanocomposite exists as relatively loose aggregates of PPy-Sb2O3 with crystallize size of 100250 nm which is observed from SEM study. The amorphous polypyrrole matrix can restrict the further growth of Sb2O3 nanocrystals and avoid their further aggregation in the chemical reaction process. According to above results, it can be summarized that, the parameter modulation of PPy in presence of Sb2O3 nPs affects not only the final morphology but also the structure of Sb2O3 nPs within the PPy-Sb2O3 nanocomposites.
Fig.8a
Fig.8b
Fig.8c
Fig.8d
Fig.8e
Fig.8a-8e SEM images of pure PPy (a), PPy-Sb2O3 nanocomposites (b,c,d), pure Sb2O3 nPs (e)
Succeeded in controlling PPy-Sb2O3 morphology, the investigation was turned to explore, the formation mechanism of the PPy-Sb2O3 morphology through characterizing the intermediates obtained in different reaction stages. The change in the surface morphology has been observed with increasing composition of Sb2O3 (25-100 wt %) in PPy-Sb2O3 nanocomposites. The complex, stringy, interconnected network is a general feature of the morphology of PPy-Sb2O3 nanocomposites. At higher (100 wt%) of nanocomposites, the connected path way become more and more dense morphology are observed due to excess doping as the PPy- Sb2O3 is approached. At this higher percentage of PPy-Sb2O3 nanocomposites; the morphology appears almost foam like with PPy-Sb2O3 network surrounded by Sb2O3. Thus Sb2O3 provides large conduction island thereby, reducing the conduction path through the nanocomposites [17].
-
Energy dispersive X-ray analysis
Fig. 9(a-e) shows the EDAX spectrum of the pure PPy, PPy-Sb2O3 (25-100%) nanocomposites and pure Sb2O3 nPs. The corresponding chemical composition is listed in Table.5. Fig.9a illustrates the element weight (%) of C, O, and S of pure PPy sample was 69.32%, 24.24% and 6.44% respectively. It is seen that C, O, S and Sb elements are detected in the PPy-Sb2O3 (25-100%) nanocomposites, which indicates that O and Sb-ions have been doped into the PPy matrix successfully. The spectrum of pure PPy, PPy-Sb2O3 (25-100%) nanocomposites and pure Sb2O3 nPs shows of the carbon molecules weight % are 69.32%, 40.66%, 30.02%, 17.87% and 14.80% which is element composition in which decreasing trend is appeared for the same element composition changes were obtained for oxygen and sulfur molecules weights, and the weight % of antimony ion are 35.72 %, 49.98
%, 62.05 % and 85.20 %, for this chemical composition increasing trend was appeared. As shown in Fig.9b an element like carbon, sulfur, oxygen and antimony of PPy-Sb2O3 nanocomposite samples which compare due to pure PPy, the element contents of carbon, oxygen and sulfur was decreased,
Antimony alone increased with increasing the concentration of Sb2O3 nanoparticles [18].
Fig.9 EDAX spectra of pure PPy (a), PPy-Sb2O3 nanocomposites (b,c,d) and pure Sb2O3 nPs (e)
Sample Name
Weight (%)
Carbon
Oxygen
Sulfur
Antimony
Total
Pure PPy
69.32
24.24
6.44
–
100
PPy- Sb2O3 (25%)
40.66
19.60
4.02
35.72
100
PPy- Sb2O3 (50%)
30.02
17.35
2.65
49.98
100
PPy- Sb2O3 (100%)
17.86
18.24
1.85
62.05
100
Pure Sb2O3
14.80
–
–
85.20
100
Table.5 Element analysis data of pure PPy, PPy-Sb2O3 nanocomposites and pure Sb2O3 nPs
The element contents of PPy-Sb2O3 (25-100%) nanocomposites show a higher Sb contents due to the formation of a large amount of Sb2O3.
-
-
Conclusion
The FTIR analysis is carriedout for pure PPy, Sb2O3 nPs and PPy-Sb2O3 (25-100%) nanocomposites systematically. The characteristics bands were observed for the corresponding materials. From the results, one can conclude that, the wavenumber region is shifted to higher values after Sb ion absorption. The results idicate that the co-
ordination bond formed between the long pair of electrons of the atom in the PPy chain with the orbit of Sb atom of Sb2O3, indicating the strength of PPy-Sb2O3 nanocomposites have been synthesized successfully and the observed shift which indicates the interaction between PPy and Sb2O3 nPs. UV- visible spectra results indicates that the absorption bands which are correspond to the transition of -*. From the tauc plot, one can conclude that the band gap energy is calculated for each case of materials. Among the materials, the band gap energy (3.46 eV) is obtained for pure PPy. The band gap is decreased with increasing Sb2O3 concentration and this indicates the PPy-Sb2O3 interactions are significantly increased by increasing the Sb2O3 concentration loading to reduce the energy level intervals. X-Ray diffraction studies suggest the crystallographic nature of the materialsand from the report, we analyze the XRD patterns of pure PPy, Sb2O3 nPs and PPy-Sb2O3 nanocomposites is in a systematic manner. The amorphous peak of pure PPy was appeared in addition to sharp peak, but the other patterns indicates the crystalinity was greatly improved the with addition of Sb2O3 form the nanocomposites. From the crystallite size calculations, the average crystallite size of PPy-Sb2O3 (25 wt%), PPy-Sb2O3 (50 wt%) and PPy-Sb2O3 (100 wt%) are 97 nm, 170 nm and
176 nm respectively. From this, one can inferred that the lowest average crystallite size is observed for PPy-Sb2O3 (25%). The strain and dislocation density calculation and the data suggest the crystallographic nature and defects of the materials. Thermogravimetric results of the pure PPy, Sb2O3 nPs and PPy-Sb2O3 (25-100%) nanocomposites suggest that thermal behavior and stability of the materials and the number of stages of decomposition may vary depending upon the materials. In this report, the lowest number of stages (2) is observed for pure PPy. The residual mass of PPy-Sb2O3 (25- 100 wt%) is increased, when the composition increase from PPy-Sb2O3 (25 wt%) to PPy-Sb2O3 (100 wt%). This is because, the increase of loading amount of Sb2O3 in the matrix of PPy, but the residual temperature is almost constant for all the cases. From the differential scanning calorimetric analysis, one can reveal the stages in which the molecules of various categories eliminating from the surface. The exothermic and endothermic peaks suggests that the polymer decomposition is found in the case of PPy-Sb2O3 nanocomposites. These kinds of analysis help us to estimate the thermal stability of pure PPy, Sb2O3 nPs and PPy-Sb2O3 (25-100%) nanocomposites. In this report, the surface morphological analyses of pure PPy, Sb2O3 nPs and PPy-Sb2O3 (25-100%) nanocomposites are carriedout successfully. Actually, the particles are not in spherical in size for the all the cases. Instead, the particles are agglomerated initially and the some square shaped particles are also found and also there are some surface modifications, due to the agglomeration of the particles, so that the core shell like structure is formed on the surface of PPy matrix and this can be seen clearly from the morphological data. From the EDAX analysis, the elemental composition of pure PPy, Sb2O3 nPs and PPy-Sb2O3(25-100 wt%) is estimated clearly. From the increasing trend of antimony, one can inferred the loading amount of Sb2O3 in the matrix of PPy. This kind of analysis helps us to gain more information about the structure and behavior of the materials.
REFERENCES
-
Li Cui, Juan Li, Xiao-gang Zhang. Materials Letters 63 (2009) 683-686.
-
Pi-Guey Su, Lin-Nan Huang. Sensors and Actuators B 123 (2007) 501-507.
-
Yu Xiea, Xiaowei Hong, Yunhua Gao, Mingjun Li, Jinmei Liu, Juan Wang, Jing Lu. Synthetic Metals 162 (2012) 677-681.
-
Komilla Suri, S. Annapoorni, R.P. Tandon, N.C. Mehra. Synthetic Metals 126 (2002) 137-142.
-
Komilla Sari, S.Annapoorni, A.K.Sarkar, R.P.Tandon. Sensors and Actuators B 81 (2002) 277-282.
-
Ze Hua Dong, Yan Li Wei, Wei Shi, Guo An Zhang. Materials Chemistry and Physics 131 (2011) 529-534.
-
Madhumita Bhaumik, Arjun Maity, V.V. Srinivasu, Maurice S. Onyango. Journal of Hazardous Materials 190 (2011) 381-390.
-
Sukanta De, Ashis Dey, S.K. De. Solid State Communications 137 (2006) 662-667.
-
Kousik Dutta, S.K. De. Solid State Communications. 140 (2006) 167171.
-
Debabrata Nandi, Arup Kumar Ghosh, Kaushik Gupta, Amitabha De, Pintu Sen, Ankan Duttachowdhury, Uday Chand Ghosh. Materials Research Bulletin 47 (2012) 20952103.
-
Wenqin Wang, Wenli Li, Ruifeng Zhang, Jianjun Wang. Synthetic Metals 160 (2010) 2255-2259.
-
Haldorai Yuvaraj, Eun Ju Park, Yeong-Soon Gal, Kwon Taek Lim. Colloids and Surfaces A: Physicochem. Eng. Aspects 313-314 (2008) 300-303.
-
Manawwer Alam, Anees A. Ansari, Mohammed Rafi Shaik, Naser M. Alandis. Arabian Journal of Chemistry (2012).
-
Emine Temizel, Esra Ayan, Mehmet S¸ enel, Hamit Erdemi, Mustafa S. Yavuz, Huseyin Kavas, Abdulhadi Baykal, Ramazan Ozturk. Materials Chemistry and Physics 131 (2011) 284-291.
-
Hamid Heydarzadeh Darzi, Saeedeh Gilani Larimi, Ghasem Najafpour Darzi. Synthetic Metals (2011).
-
S.Y. Chew, Z.P. Guo, J.Z. Wang, J. Chen, P. Munroe, S.H. Ng, L. Zhao, H.K. Liu. Electrochemistry Communications 9 (2007) 941946.
-
C. Lai, G.R. Li, Y.Y. Dou, X.P. Gao. Electrochimica Acta 55 (2010) 4567-4572.
-
Madhumita Bhaumika, Taile Yvonne Leswifia, Arjun Maity,
V.V. Srinivasu, Maurice S. Onyango. Journal of Hazardous Materials 186 (2011) 150159.